the Creative Commons Attribution 4.0 License.
the Creative Commons Attribution 4.0 License.
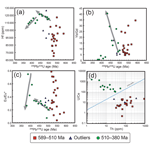
Unraveling the origins and P-T-t evolution of the allochthonous Sobrado unit (Órdenes Complex, NW Spain) using combined U–Pb titanite, monazite and zircon geochronology and rare-earth element (REE) geochemistry
José Manuel Benítez-Pérez
Pedro Castiñeiras
Juan Gómez-Barreiro
José R. Martínez Catalán
Andrew Kylander-Clark
Robert Holdsworth
The Sobrado unit, within the upper part of the Órdenes Complex (NW Spain) represents an allochthonous tectonic slice of exhumed high-grade metamorphic rocks formed during a complex sequence of orogenic processes in the middle to lower crust. In order to constrain those processes, U–Pb geochronology and rare-earth element (REE) analyses of accessory minerals in migmatitic paragneiss (monazite, zircon) and mylonitic amphibolites (titanite) were conducted using laser ablation split stream inductively coupled plasma mass spectrometry (LASS-ICP-MS). The youngest metamorphic zircon age obtained coincides with a Middle Devonian concordia monazite age (∼380 Ma) and is interpreted to represent the minimum age of the Sobrado high-P granulite facies metamorphism that occurred during the early stages of the Variscan orogeny. Metamorphic titanite from the mylonitic amphibolites yield a Late Devonian age (∼365 Ma) and track the progressive exhumation of the Sobrado unit. In zircon, cathodoluminescence images and REE analyses allow two aliquots with different origins in the paragneiss to be distinguished. An Early Ordovician age (∼490 Ma) was obtained for metamorphic zircons, although with a large dispersion, related to the evolution of the rock. This age is considered to mark the onset of granulite facies metamorphism in the Sobrado unit under intermediate-P conditions, and related to intrusive magmatism and coeval burial in a magmatic arc setting. A maximum depositional age for the Sobrado unit is established in the late Cambrian (∼511 Ma). The zircon dataset also record several inherited populations. The youngest cogenetic set of zircons yields crystallization ages of 546 and 526 Ma which are thought to be related to the peri-Gondwanan magmatic arc. The additional presence of inherited zircons older than 1000 Ma is interpreted as suggesting a West African Craton provenance.
- Article
(13079 KB) - Full-text XML
- BibTeX
- EndNote
Zircon, monazite and titanite are accessory mineral phases found in rocks with a very wide range of compositions. These minerals can resist numerous sedimentary, igneous and metamorphic events across a wide range of temperatures, pressures and strains, even when fluids are present. Frequently, compositional domains can be defined in these minerals that record changes in different parameters (Storey et al., 2007; Castiñeiras et al., 2010; Stübner et al., 2014; Hacker et al., 2015; Stearns et al., 2016; Stipska et al., 2016). These minerals additionally provide several closed decay chains or disintegration systems (238U → 206Pb, 235U → 207Pb y 232Th → 208Pb), because they hold variable concentrations of uranium (U) and/or thorium (Th) in their crystal lattices. Such variations in concentration allow accurate dating using microscopic-scale analysis (tens of micrometers).
Titanite is stable in metabasites across a wide range of metamorphic conditions (Spear, 1981; Frost et al., 2000) and is able to record metamorphic and deformational events (Franz and Spear, 1985; Verts and Frost, 1996; Rubatto and Hermann, 2001; Spencer et al., 2013; Stearns et al., 2015, 2016). The titanite U∕Pb system is a widely used geochronometer for deformation events in granulite–amphibolite facies rocks (Spear, 1981; Cherniak, 2006; Harlov et al., 2006). Monazite is common in amphibolite and higher-grade facies. Zoning in this mineral can have an igneous or metamorphic origin (DeWolf et al., 1993; Hawkins and Bowring, 1997; Zhu et al., 1997; Spear and Pyle, 2002). The crystallization stages seen in zoned monazite, with changes in Y, Ca, Si, Sr, Ba, rare-earth elements (REEs), U and Th, has been linked to certain metamorphic reactions (Kohn and Malloy, 2004; Corrie and Kohn, 2008) or deformation process (Terry and Hamilton, 2000). Zircon survives the majority of magmatic, metamorphic and erosive terrestrial processes. Cathodoluminescence analysis of zircon zoning patterns allows a large variety of reactions to be distinguished and can clarify the petrogenetic evolution (Corfu et al., 2003). Th∕U ratios can also be used to separate zircon based on their igneous or metamorphic origin (Hoskin and Ireland, 2000; Möller et al., 2002; Hokada and Harley, 2004; Hoskin, 2005). REE abundances can also be used as a qualitative petrological indicator (Belousova et al., 2002). Heavy rare-earth elements (HREEs) are preferentially incorporated into zircon compared to light rare-earth elements (LREEs). Hence, the normalized HREE slope can be used to interpret whether a zircon crystallized or recrystallized when garnet and xenotime (YPO4) were present, because these minerals also preferentially assimilate HREE in the lattice (Hoskin and Ireland, 2000; Rubatto, 2002; Hermann and Rubatto, 2003; Rubatto et al., 2009).
The events recorded in individual grains can be radiometrically dated employing combined laser ablation analyses and cathodoluminescence (CL) images in zircons (Corfu et al., 2003) and compositional maps obtained using electron microprobe (EMPA) in monazite (Gonçalves et al., 2005; Williams et al., 2007) to recognize different growth zones. The chemical analysis, especially REE, links the development of growth zones to specific metamorphic or deformative events (Frost et al., 2000; Rubatto, 2002; Whitehouse and Platt, 2003; Zheng et al., 2007; Chen et al., 2010; Gagnevin and Daly, 2010; Holder et al., 2015;). Simultaneous geochronology and REE data are a powerful tool in the interpretation of ages – this is known as REE-assisted geochronology (Castiñeiras et al., 2010).
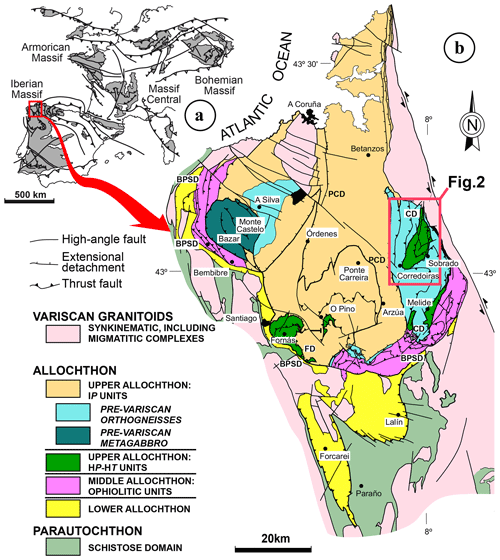
Figure 1(a) Simplified map of the Variscan orogen in Europe with the location of the Órdenes Complex. (b) Map of the Órdenes Complex with indication of the main units and tectonic contacts. Extensional detachments are labeled: BPSD: Bembibre–Pico Sacro system; CD: Corredoiras; FD: Fornás; PCD: Ponte Carreira. Figure 2 location is indicated.
This methodology has been applied to selected samples of the Sobrado unit (Fig. 1), which forms part of the allochthonous complexes of NW Spain and one where the structural and metamorphic evolution is rather well known (de Pablo Maciá et al., 1984; Díaz García et al., 1999; Arenas and Martínez Catalán, 2002; Benítez-Pérez, 2017). This unit occurs in the upper allochthon of the Órdenes Complex and represents a tectonic slice of exhumed ultramafics, eclogites, high-P granulites, amphibolites and migmatitic paragneiss derived from a peri-Gondwanan terrane. It evolved along a complex sequence of geological processes involving Cambrian–Ordovician rifting with voluminous bimodal plutonism, nearly contemporaneous granulite facies metamorphism of intermediate-P, early Variscan subduction and subsequent exhumation by ductile thrusting during Variscan collision with the external margin of northern Gondwana.
Previous geochronological data on the Sobrado unit include U–Pb ages from four samples (Fernández-Suárez et al., 2002, 2007). A middle Cambrian protolith age of a gabbro and a Middle Devonian metamorphic age of a high-P basic granulite supposedly derived from the same gabbro were obtained by zircon dating. Zircon in a migmatitic, mylonitized paragneiss yielded discordant ages with an Early Ordovician lower intercept, while monazite dating provided Cambrian–Ordovician ages in another migmatitic, mylonitized paragneiss. This new study aims at constraining the metamorphic evolution of the unit including dating a migmatitic paragneiss, as previous data missed the early Variscan ages found in intercalated high-P granulites, and also an amphibolite, which could date advanced stages of exhumation. Monazite and zircon ages of paragneiss and titanite ages of amphibolites taken from separate but presently adjacent tectonic slices of the high-P/high-T (HP-HT) of the Sobrado unit are compared and interpreted using REE-assisted geochronology. This sheds new light upon the possible origin, ages and relationships between the regional foliation development and the partial melting processes that have occurred in this and equivalent units of the NW Iberian upper allochthon.
The allochthonous complexes in NW Spain are remnants of a huge nappe stack preserved as klippen in the core of late Variscan synforms. They consist of units mostly of peri-Gondwanan derivation, which can be classified in three groups based on their structural position in the tectonic pile and origin: the upper, middle and lower allochthons (Fig. 1).
The upper allochthon is a piece of the northern margin of Gondwana detached and drifted away during the Cambrian–Ordovician opening of the Rheic Ocean. The middle allochthon is formed by lithospheric pieces of oceanic affinity or oceanic supracrustal sequences that formed part of the Rheic oceanic realm and are often referred to as ophiolitic units. The lower allochthon derives from distal parts of the Gondwanan continental margin.
The allochthon units are separated from the Iberian autochthon by a series of kilometer-scale imbricated sheets, known as the parautochthon (Ribeiro et al., 1990), or Schistose Domain in Galicia (NW Spain), consisting of a set of Paleozoic metasedimentary and volcanic rocks. The parautochthon has stratigraphic and igneous affinities with the Iberian autochthon of the Central Iberian Zone, and no ophiolites occur between them. For these reasons, it is interpreted as a distal part of the Gondwanan continental margin (Farias et al., 1987; Dias da Silva et al., 2014).
The allochthonous units are regarded as a stack of Variscan thrust sheets with associated tectonic fabrics and metamorphic events. Due to the “piggy-back” nature of the sequence, the structurally higher units are thought to represent the furthest traveled paleogeographic domains. Recumbent folds, thrusts and extensional detachments formed during the Variscan collision are found in all three allochthonous units (Martínez Catalán et al., 1999; Gómez-Barreiro et al., 2007).
Maximum sedimentation ages obtained from the study of detrital zircon carried out in metasediments from the upper allochthon can be estimated between 530 and 510 Ma (e.g., Fuenlabrada et al., 2010). Intrusive rocks in the upper allochthon have been dated between 520 and 490 Ma and are associated with the development of a magmatic arc which evolves into an extensional scenario that ended with the opening of the Rheic Ocean (Peucat et al., 1990; Ordóñez Casado, 1998; Abati et al., 1999, 2007; Fernández-Suárez et al., 2007; Castiñeiras et al., 2010). Two high-P/high-T metamorphic events have been recognized in this unit. The oldest one has yielded ages of 490–480 Ma (Kuijper 1979; Peucat et al. 1990; Abati et al., 1999, 2007; Fernández-Suárez et al., 2002) and the youngest one has been dated approximately between 405 and 390 Ma (Santos Zalduegui et al., 1996; Ordóñez Casado et al., 2001; Fernández-Suárez et al., 2007). In the middle allochthon, crystallization ages vary between 390 and 375 Ma (Peucat et al., 1990; Dallmeyer et al., 1991, 1997), and ages from 375 to 365 Ma have been related to continental subduction (Santos Zalduegui et al., 1995; Abati et al., 2010). Thrust wedge collapse, in the middle and lower allochthonous units, is thought to have happened between 390 and 365 Ma, followed by a collision in the internal zones around 365–330 Ma, causing further folding and thrusts (Dallmeyer et al., 1997; Martínez Catalán et al., 2009). Afterwards, there was another extensional collapse phase until 315 Ma, followed by a final phase of shortening and folding up until approximately 305 Ma related to the regional oroclinal bending in Spain (Aerden, 2004; Martínez Catalán, 2011, 2012; Álvarez-Valero et al., 2014).
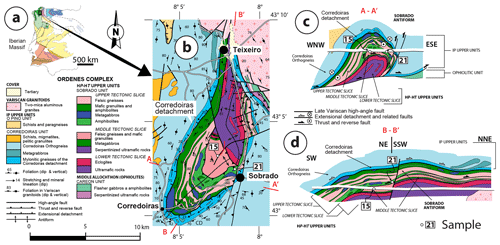
Figure 2Geological map of the study area and location of the samples, modified from Arenas and Martínez Catalán (2002): (a) location of the Órdenes Complex within the Iberian massif. (b) Sobrado unit map, indicating units and tectonic slices. (c) Cross-section in the WNW–ESE direction and (d) SW–NE and SSW–NNE directions of the Sobrado antiform. Sample locations are indicated.
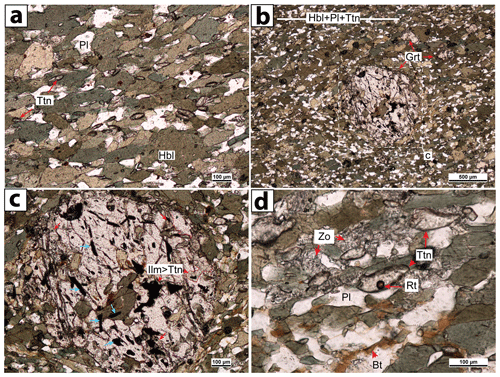
Figure 3Fine-grained amphibolites (sample JBP-71-21) from the basal mylonitic band in the Sobrado upper tectonic slice. (a) Hornblende–plagioclase mixtures and titanite with preferred orientation, defining the mylonitic fabric. (b) Main fabric surrounding a garnet porphyroclast. Note that titanite is the Ti phase in the mylonitic fabric but ilmenite grains have been preserved inside the garnet. The position of panel (c) is indicated by a dashed white square. (c) Garnet inclusions from panel (b) where ilmenite inclusions show a progressive breakdown into titanite (Ilm < Ttn). Note ilmenite inclusions inside pristine garnet areas show no evidence of transformation. (d) Inclusions of rutile inside titanite and partial transformation of plagioclase into zoisite. All micrographs are in plane-polarized light (PPL). (Mineral abbreviations are after Whitney and Evans, 2010; Hbl: hornblende, Ttn: titanite, Ilm: ilmenite, Rt: rutile, Pl: plagioclase, Zo: zoisite, Grt: garnet.)
The upper allochthon is further subdivided into high-P/high-T and intermediate-P units (Gómez Barreiro et al., 2007). The present study focuses on two of the high-P/high-T upper units (Figs. 1 and 2). The origin of the high-P event recorded in these units is controversial but might reflect the accretion of the units into the continental part of northern Gondwana, during the Early–Middle Devonian (400–390 Ma; Ballèvre et al., 2014).
The Sobrado antiform consists of three tectonic slices bounded by two extensional detachments (Figs. 1 and 2). The lower tectonic slice comprises highly serpentinized ultramafic rocks with interlayered metabasite units. The metabasites include eclogites (Omp + Grt + Qtz + Rt ± Ky and Zo, mineral abbreviations according to Whitney and Evans, 2010) and related clinopyroxene-garnet rocks without primary plagioclase (Cpx + Grt + Qtz + Rt ± Zo), as well as other types of rocks derived from the retrogression and mylonitization of the early high-P stages. The intermediate tectonic slice is made up of migmatitic felsic gneiss (mainly paragneiss), with frequent inclusions of high-P granulites (Cpx + Grt + Pl + Qtz + Rt ± Ky). Remnants of igneous protoliths are not preserved either in the lower or intermediate tectonic slices. The upper tectonic slice, however, contains migmatitic felsic gneiss and mafic layers derived from deformed and recrystallized gabbros with locally preserved relict igneous textures, reaching high-P granulite facies conditions. The progressive transformation from gabbros to high-P granulites (Na–Di + Grt + Pl + Qtz + Rt) has occurred in a series of different stages with a metamorphic peak at 13–17 kbar and 660–770 ∘C (Arenas and Martínez Catalán, 2002).
The metamorphic evolution of the Sobrado unit as described in the literature indicates that felsic gneiss underwent differing degrees of partial melting after the metabasites reached their peak pressure. Consequently, the felsic gneiss is thought to have developed a regional foliation under amphibolite facies conditions, as did the amphibolic gneiss, “flaser” amphibolites and fine-grained amphibolites. This metamorphic evolution is described by Arenas and Martínez Catalán (2002) as a clockwise P–T path, with a metamorphic peak of, at least, 15 kbar and >800 ∘C, followed by an isothermal decompression. This trajectory is interpreted to result from gravitational collapse of an overthickened orogenic wedge (Gómez-Barreiro et al., 2007; Ballèvre et al., 2014). Although some regional structures, such as the Fornás detachment (FD, Fig. 1; Gómez-Barreiro et al., 2007; Álvarez-Valero et al., 2014) or the Corredoiras detachment (CD, Fig. 1; Díaz García et al., 1999), have been related to this gravitational readjustment, no study has dealt with the development of the extensional fabrics in any detail. Overall, it is thought that the extensional flow has generated a pervasive thinning of the orogenic pile and that the preserved sequence of tectonic slices is strongly condensed.
3.1 Selected samples
Two representative samples (JBP-71-15A and JBP-71-21) were selected from two structurally separate but currently adjacent parts of the high-P/high-T Sobrado unit, within the Órdenes Complex, for laser ablation (laser ablation split stream; LASS) analyses, including U–Pb geochronology and REE determinations. The samples locations are presented in Fig. 2.
Sample JBP-71-21 is a mylonitic fine-grained amphibolite, without any preserved igneous relicts. Fine-grained amphibolites represent an advanced stage in the mylonitization of the metabasites. They appear as relatively thin layers inside the mafic rocks and dominate in a thick mylonitic layer (300–700 m; Fig. 2; Arenas and Martínez Catalán, 2002; Benítez Pérez, 2017) located at the base of the upper tectonic slice. The sample comprises Hbl + Pl + Grt ± Cpx + Bt ± Rt ± Ttn ± Ilm ± Zo ± Qtz (Fig. 3). Mylonitic foliation and lineation are defined by amphibole and plagioclase (Fig. 3). Garnet appears as subrounded porphyroclasts partially resorbed (Fig. 3). Titanite grains are parallel to the foliation and in textural equilibrium with plagioclase and amphibole. Rutile and ilmenite are always hosted as inclusions both in titanite and/or garnet (Fig. 3c, d).
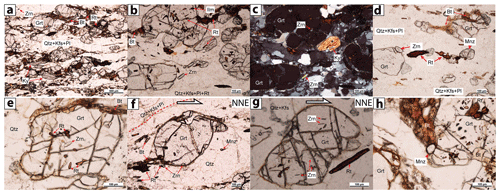
Figure 4Granulite facies migmatitic paragneiss from the Sobrado middle tectonic slice (Fig. 2). (a) General microstructure where leucosome bands and preferred orientation of elongated garnets, biotite, rutile and kyanite define the foliation. (b) Zircon elongated grain in recrystallized leucosome domain. Main inclusions in garnet include rutile, ilmenite and zircon (PPL) and (c) cross-polarized light (CPL). (d) Monazite and zircon subrounded grain in the leucosome domain. (e) Subrounded elongated grain of zircon included in a garnet. (f) Plastically deformed garnet (sigmoidal grain) in a leucosome. Zircon and monazite are found in the quartz-rich area around the garnet. (g) Sigmoidal garnet in a leucosome. Inclusions of prismatic and bipyramidal zircons and rutile are observed. (h) Monazite grain in a garnet pressure shadow with biotite. Ky: kyanite, Bt: biotite, Qtz: quartz, Kfs: K-feldspar, Pl: plagioclase, Rt: rutile, Zrn: zircon, Mnz: monazite, Grt: garnet.
Sample JBP-71-15A is a granulite facies migmatitic paragneiss from the underlying intermediate tectonic slice. It comprises Qtz + Pl + Grt + Kfs + Ky + Bt + Ilm + Rt and shows microscopic-scale textural evidence of partial melting. Temperature and pressure estimation ranges are 750–850 ∘C and 11–16 kbar for the anatectic fabric (Benítez-Pérez, 2017). Leucocratic domains with Qtz, Kfs and Pl, with evidence of plastic deformation define the foliation and Bt, Ky, Rt and Grt define linear aggregates resulting in a pervasive lineation (Fig. 4). Along the strained leucosomes, garnets show evidence of plastic deformation (Benítez Pérez, 2017) like (Fig. 4a, f, g): sigmoidal, dumbbell-shaped grains and pinch-and-swell microstructures (Ji and Martignole, 1994; Kleinschrot and Duyster, 2002; Passchier and Trouw, 2005). Zircon and monazite are found in different microtextural settings. Small elongated prims of zircon are always found shielded within garnets (Fig. 4g), while relatively larger zircon grains with irregular, elongated, subrounded shapes, appear across the fabric in leucosomes, biotite aggregates and even within kyanite crystals (Fig. 4a, b, c, d, f). In a few cases, elongated/subrounded zircons have been found as inclusions in garnets (Fig. 4e). Monazite grains show elongated to subrounded grains located in Qtz–Kfs–Pl–Bt domains, which define the main foliation of the rock (Fig. 4d, f, h).
3.2 Sample preparation
Sample preparation was carried out at the laboratories of the Universidad Complutense (Madrid). The rocks were crushed, pulverized and sieved to achieve a 0.1–0.5 mm grain size. Heavy minerals were concentrated using a Wilfley™ table. The non-magnetic minerals from this heavy fraction are then separated using a Frantz isodynamic magnetic separator. A final concentrated fraction is obtained using heavy liquids (methylene iodide, CH2I2).
Zircon (translucent, colorless or light brown), monazite (yellow) and titanite (colorless) grains are selected by handpicking, according to their external morphology viewed under a binocular microscope. All the grains collected were arranged separately in parallel rows, mounted on glass slide with a double-sided adhesive and set in epoxy resin. After the resin was cured, the surface was eroded using a wet abrasive silicon carbide abrasive paper (4000 grit) and polished with 0.3 µm aluminum oxide. The surface was then coated with gold, to avoid charging problems under the scanning electron microscope (SEM). Prior to isotopic analysis, CL images of zircon grains were taken on a JEOL JSM-820 SEM, and compositional maps of monazite grains were created on a JEOL Superprobe JXA-8900M microprobe (National Center for Electron Microscopy, Madrid). Secondary electron (SE) images were also taken to determine the exact location of the spots and identify the internal structure and presence of inclusions and defects in zircon, monazite and titanite grains.
3.3 Mineral description
Titanite grains are generally rounded, with an average grain size of 100 µm and irregular morphologies. Their secondary electron images reveal homogeneous compositions and the presence of solid inclusions. This grain size permits large spatial resolution analyses (50 µm) to be carried out. Monazite grains have a more variable grain size distribution, with an average of 60–70 µm. Their habit is irregular and they usually show rounded morphologies or broken grains. We carried out La, Th, Y, U and Nd compositional maps for every monazite grain in order to discover a compositional zonation that could be attributed to different growth events. Thorium zoning is the one that developed better and was taken into account to select the spots for isotopic analysis (Fig. 5), yet it never exceeds 30 % of the grain. Several spots were analyzed in monazite crystals with the greatest compositional contrasts to determine if they really represented different growth stages in the monazite grains.
Zircon grains from the paragneiss usually have scarce mineral inclusions and they can display a wide variety of morphologies, including irregular and subrounded shapes typical of metamorphic zircon, pristine elongated dipyramidal prisms interpreted as igneous in origin and equigranular grains with abrasion signs with a probable detrital origin. Their length-to-width ratios vary between 3:1 and 2:1. Cathodoluminescence images (Fig. 6) are useful to relate the crystallization of parts of zircon crystals to specific igneous, metamorphic or deformational events (Corfu et al., 2003; Nasdala et al., 2003; Zeck et al., 2004). It is common to image a homogeneous xenocrystic core in zircon grains and even a less luminescent mantle in some grains (grain numbers 5, 6). The core aspect is mainly rounded, with irregular or angular shapes. In most of the zircon grains, the internal parts of the grains display an oscillatory zoning (grain numbers 33, 71), with different thickness, although in some cases, this zoning is faint (grain number 26). There are several grains with sector zones (grain numbers 26, 27) parallel to the zircon c axis (Watson and Yan Liang, 1995) and even one case of soccer-ball zoning (grain number 82). The zoning usually appears to be partially truncated and surrounded by a discontinuous poorly luminescent rim (grain numbers 20, 79).
3.4 Analytical techniques
, REE and Hf analyses of zircon, titanite and monazite were carried out using the laser ablation split stream (LASS) at the University of California at Santa Barbara (UCSB). The samples were ablated using a Photon Machines 193 nm ArF excimer ultraviolet laser with a HelEx ablation cell coupled to a Nu Instruments Plasma high-resolution multi-collector inductively coupled plasma mass spectrometer (MC-ICP-MS) and either a Nu Instruments AttoM high-resolution single-collector ICP or an Agilent 7700S quadrupole ICP-MS (Kylander-Clark et al., 2013). This installation allows the simultaneous isotopic and compositional (REE) analysis to be carried out. The laser spot diameter was 20 µm for zircon, 7–10 µm for monazite (Košler et al., 2001) and 50 µm for titanite (Stearns et al., 2016), resulting in pit depths between 6 µm for monazite and 30 µm for titanite. The laser has a fluence of ∼1 J cm−2 and was fired twice to remove common Pb from the sample surface. This material was allowed to wash out for 15 s, prior to the material being ablated at 3 Hz for 20 s for analysis. On the ICP-MS, the masses 204Pb + Hg, 206Pb, 207Pb and 208Pb were measured using ion counters, and the masses 232Th and 238U were measured using Faraday detectors.
The standardization for monazite was carried out using sample 44069 (Aleinikoff et al., 2006) as the primary reference material (RM), whereas the Bananeira sample was employed as primary RM for trace element corrections (Kylander-Clark et al., 2013; Palin et al., 2013). Additionally, FC-1 (Horstwood et al., 2003), Trebilcock (Tomascak et al., 1996) and Bananeira were also used as secondary monazite RM, allowing 206Pb∕238U ages to be within 2 % of their accepted values. U–Pb proportions in titanite were corrected using Bear Laken (Aleinikoff et al., 2007) and Y1710C5 (Spencer et al., 2013) as RM. Overall, the 91500 sample (Wieldenbeck et al., 1995) and GJI (Jackson et al., 2004) were used as RM for zircon, both for isotopic composition and trace element calibrations. Radiogenic lead versus common lead (207Pb∕206Pb) measurements require up to 2 % additional external error attributable either to variation count statistics, or ablation signal stability (Spencer et al., 2013; Hacker et al., 2015b). These external errors were incorporated into the data in the experiments.
The Iolite plug-in version 2.5 (Paton et al., 2011) from the Wavemetrics Igor Pro software was used to improve and reduce the analyses (Hacker et al., 2015). The isotopic ratios 238U∕206Pb and 207Pb∕206Pb for each analysis were plotted on Tera–Wasserburg diagrams (Tera and Wasserburg, 1972) using Isoplot and Topsoil programs (Ludwig, 2012; Zeringue et al., 2014). All date uncertainties are reported at the 95 % confidence interval, assuming a Gaussian distribution of measurement errors. Zircon, titanite and monazite REE analyses were normalized against the McDonough and Sun (1995) chondrite values.
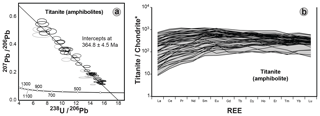
Figure 7(a) Tera–Wasserburg diagram showing distribution of analyzed titanite (n=51) from Sobrado amphibolite (JBP-71-21). The rejected analyses are represented by gray ellipses. The ellipses represent the 207Pb∕206Pb and 238U∕206Pb errors (± 2σ). (b) Chondrite-normalized REE patterns for the same titanite.
4.1 Titanite (amphibolite, upper tectonic slice)
Overall, 51 titanite analyses were projected onto a Tera–Wasserburg concordia plot (Fig. 7a). After a preliminary evaluation, 12 analyses were rejected due to either high common Pb or high discordance (>10 %) and were considered no further (Table 1). The remaining analyses define a Pb∕U semi-total isochron between the common or initial Pb (207Pb) and radiogenic Pb (206Pb) (Ludwig, 1998). The good fit of the isochron confirms the chemical homogeneity of the data (Stearns et al., 2016) and it intercepts the concordia at 364.8±4.5 Ma (2σ). Titanite chondrite-normalized REE analyses are detailed in Table 1 and are shown in Fig. 7b. Titanite REE patterns are convex upwards, relatively flat, with slight LREE depletions versus HREE with respect to chondrite. They generally lack a europium anomaly (Eu∗), but some analyses show a non-distinctive positive or negative anomaly.
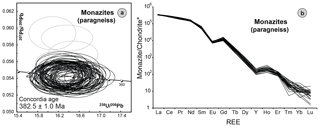
Figure 8(a) Tera–Wasserburg diagram showing distribution of analyzed monazite (n=76) from Sobrado paragneiss (JBP-71-15). The rejected analyses are represented by gray ellipses. The ellipses represent the 207Pb∕206Pb and 238U∕206Pb errors (± 2σ). (b) Chondrite-normalized REE patterns for the same monazite.
4.2 Monazite (paragneiss, middle tectonic slice)
For monazite geochronology, we used the thorium zoning in monazite grains to select the analytic spots. As shown in Fig. 5, there are no significant age differences between spots or zones with different Th chemical concentrations in a single grain. The obtained REE patterns are also very similar, and the mismatch at the HREE is probably due to either uncertainties in measurement because of the lower counts or interference effects of intermediate rare-earth oxides (Holder et al., 2015).
Data from 76 monazite analyses are shown in Table 2 and displayed using a Tera–Wasserburg concordia plot (Fig. 8a). Four of these analyses, not related to chemical zoning, were discarded due to common Pb loss and were considered no further. The remaining analyses form a single population (mean square of weighted deviation; MSWD = 0.48) centered on a concordia age of 382.5 ± 1.0 Ma (2σ). Monazite geochemistry is shown in Fig. 8b. REE patterns analyzed show an LREE enrichment, HREE depletion and negative Y anomalies with respect to chondrite with little variation within or between samples.
4.3 U–Pb zircon (paragneiss, middle tectonic slice)
Overall, 83 analyses were performed on 80 zircon grains from the Sobrado paragneiss (Table 3). In a preliminary assessment of the data, two analyses were rejected due to analytical errors (grain numbers 8 and 36). Additionally, 23 analyses yielded a discordance higher than 10 % and will not be further considered. The remaining 58 zircon analyses are shown on a Wetherill concordia plot (Fig. 9a). The 207Pb∕206Pb ages older than 1000 Ma are presented in a probability density plot (Fig. 9b). Most of the old ages are distributed between 1880 and 2200 Ma, with two peaks at 2030 and 2100 Ma. Three ages are older, around 2600 Ma, and there are also two analyses around 1300 Ma. The 206Pb∕238U ages younger than 1000 Ma are plotted on a Tera–Wasserburg concordia plot (Fig. 10a) and a probability density plot (Fig. 10b). The data are continuously distributed between 589 and 380 Ma and attending to their CL texture, ages from 589 to 510 Ma are obtained mainly from internal areas with oscillatory or sector zoning (e.g., 33, 27 and 62; see Fig. 6), whereas ages from 510 to 380 Ma correspond to discordant rims that have homogeneous CL signal, except for some grains (e.g., 10, 11 and 26; see Fig. 6) that are cores with oscillatory or sector zoning.
4.4 REE zircon (paragneiss, middle tectonic slice)
The chondrite-normalized REE patterns of the zircons with ages older than 600 Ma are shown in Fig. 11. In general, this group has REE patterns with a pronounced fractionation from light to heavy REE and two anomalies in Ce (positive) and in Eu (negative). There are only three analyses that diverge from this trend and show a flat HREE pattern.
For the younger analyses, the chondrite-normalized REE patterns have different features depending on the age (Fig. 12). The oldest ages (589–510 Ma) show a tight HREE fractionation, variable Eu anomaly, and a pronounced Ce anomaly. The youngest ages (510–380 Ma) has a variable HREE slope, whereas the Eu anomaly is more regular, and the Ce anomaly is less marked.
When we plot age versus Hf, Yb∕Gd and (Fig. 13a–c), the group of oldest ages (589–510 Ma) does not define any apparent trend. In sharp contrast, in the 500–380 Ma group it is remarkable not only the good correlation between age and composition but also the divergent evolution depending of the age (gray arrows). Hafnium, Yb∕Gd and increase from 500 to ∼430 Ma, while there is a striking decrease from ∼420 to 380 Ma. Finally, we have used the graph proposed by Bacon et al. (2012) to discriminate between metamorphic and igneous zircon.
5.1 Zircon geochronology: inheritance and age of magmatism
The presence of zircon inclusions shielded in garnets (e.g., Fig. 4e and g) is compatible with the preservation of magmatic, metamorphic or detrital inheritances during the syn-kinematic partial melting recorded in these rocks (Benítez Pérez, 2017). As shown in Fig. 12, the majority of analyzed zircon grains older than 600 Ma have fractionated REE patterns that are characteristic of igneous zircon (Hoskin and Schaltegger, 2003; Whitehouse and Platt, 2003; Hanchar and Westrenen, 2007; Grimes et al., 2015). Only three analyses show a flat HREE pattern that can be related to the presence of garnet and interpreted as metamorphic zircon. The provenance of these zircon grains older than 600 Ma in the Sobrado migmatitic paragneiss (Fig. 9b), is probably similar to those reported in the intermediate-P units of NW Spain upper allochthons like the Betanzos unit (Fuenlabrada et al., 2010) and Cariño gneiss (Albert et al., 2015). We obtained two Mesoproterozoic ages, between 1.2 and 1.4 Ga. Similar ages are also found in the parautochthonous (Díez Fernández et al., 2012), in the basal allochthonous units (Díez Fernández et al., 2010) and, to a lesser extent, in the intermediate-P units of NW Spain (Albert et al., 2015). These inherited zircons, although scarce (Fernández-Suárez et al., 2003), likely have their origin in rocks derived from Saharan and Arabian–Nubian cratons, and presumably transported during the Cadomian orogeny (e.g., Martínez Catalán et al., 2004). Paleoproterozoic populations range from 1.8 to 2.2 Ga, clustered at 2.1 Ga, are also common in the allochthonous complexes (Fernández-Suárez et al., 2003) and their origin likely involves materials generated or reworked during the Eburnean orogeny (e.g., Egal et al., 2002; Ennih and Liegeois, 2008) from the West African Craton (Peucat et al., 2005). Finally, the Archean population in the Sobrado paragneiss ranges from 2.5 to 2.8 Ga, and it is likely related to intrusive events in the western Reguibat Shield (Schofield et al., 2012) and the northern part of the West African Craton (Albert et al., 2015), with some reworking processes of juvenile rocks formed at approximately 3.0 Ga (Potrel et al., 1998).
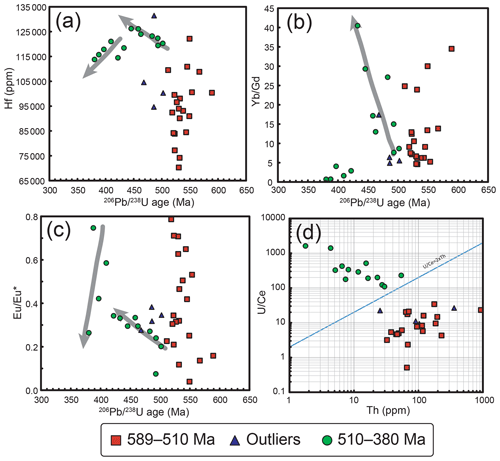
Figure 13(a) Hafnium versus age, (b) Yb∕Gd versus age, (c) versus age and (d) U∕Ce versus Th for zircon analyses between 589 and 380 Ma.
Based on CL images, ages and zircon composition, we can determine that the youngest zircon with magmatic origin is grain number 34 (Fig. 6). The number obtained in this grain is comparable to other maximum depositional ages obtained from similar units in the NW Spain allochthonous complexes, such as the intermediate-P Betanzos uppermost unit (530–510 Ma; Fuenlabrada et al., 2010) and the intermediate-P Cariño uppermost unit (∼510 Ma; Albert et al., 2015).
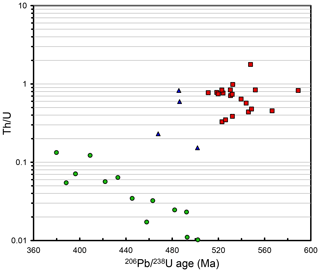
Figure 14Th∕U ratio versus 206Pb∕238U ages for the zircon analyses from 589 to 380 Ma. Analysis 63 (510 Ma) is not represented as it has an anomalous value (6.59).
In order to get more insight into the meaning of the data younger than 600 Ma, we have plotted these ages versus the Th∕U ratios (Fig. 14). Analyses that yielded ages between 589 and 510 Ma cluster together with Th∕U values higher than 0.3. The REE patterns displayed in this cluster are consistent with a magmatic origin. In the age versus Hf Yb∕Gd and plots for this group (Fig. 13a–c), the absence of a trend suggests that the different zircon grains are not connected by a fractional crystallization process (e.g., Barth and Wooden, 2010). In the graph proposed by Bacon et al. (2012) to discriminate between metamorphic and igneous zircon, zircons with ages between 589 and 510 Ma are plotted in the igneous zircon field (Fig. 13d).
From the first group of data between 589 and 510 Ma, we can extract two ages: 546 ± 5 Ma out of six analyses, and 526 ± 3 Ma from 14 analyses (Fig. 15). These magmatic ages are also recognized in other well-characterized high-P/high-T units of the allochthonous complexes (Peucat et al., 1990; Santos Zalduegui et al., 2002; Castiñeiras et al., 2010), and they are related to a magmatic arc creation around the periphery of Gondwana (Abati et al., 1999, 2007).
5.2 Evolution of metamorphism of the Sobrado migmatitic paragneiss based on zircon composition
Extracting ages from a dataset where the data are evenly distributed from 500 to 380 Ma is a challenging task. When such smear in the age sorting happens, if we can rule out analytical error, we have three possibilities (e.g., Castiñeiras et al., 2010), namely (i) the correct age is the youngest and the dispersion is related to zircon inheritance, (ii) the real age is the oldest and the spread is caused by lead loss, or (iii) the age range is recording some kind of protracted geological event. Taking into account the CL texture of the youngest spots, we can remove the first possibility as the majority of analyses were performed in zircon rims. Furthermore, maximum depositional ages obtained from similar units from the allochthonous complexes preclude their interpretation as inheritance. The second possibility is plausible, considering the complex metamorphic evolution and the high grade attained by these rocks. However, a young lead loss episode should have affected also the inherited zircon ages, and the presence of various old age peaks (e.g., ∼2000, 546 and 536 Ma) suggests that they did not experienced lead loss. However, we can argue that limited lead loss occurred in some grains that have similar composition to the 589–510 Ma group (Th∕U > 0.15) but younger ages (between 502 and 468 Ma). This group of outlier analyses (nos. 10, 11, 26 and 63) could have experienced a decoupling between their actual age and their composition (e.g., Flowers et al., 2012). Finally, we favor the last option when we take into account the strong correlation observed between age and zircon composition (Figs. 13a–c and 14).
This group of young analyses defines a trend with negative correlation from 500 to 380 Ma and Th∕U values from 0.01 to 0.13. This correlation between the age and the composition of zircon suggests that the age dispersion is related to an actual geological process and is not caused by lead loss. Furthermore, even though the Th∕U ratio shows a consistent evolution from 510 to 380 Ma, the rest of the proxies considered (Hf, Yb∕Gd and , Fig. 13a–c) point to a two-stage evolution, and the characteristics of the REE patterns are compatible with a metamorphic origin (Chen et al., 2009, 2010; Rubatto et al., 2009; Peters et al., 2013; Stipska et al., 2016).
This scenario is congruent with the presence of two metamorphic events in the HP-HT units: one at ∼490–480 Ma and the other at ∼390–380 Ma (e.g., Fernández-Suárez et al., 2002, 2007). The increase in Yb∕Gd values (Fig. 13b), related to the slope of the HREE, in zircons aged from 502 to 430 Ma is congruent with a higher availability of HREE in the rock. As garnet is the most important HREE reservoir in metamorphic rocks, we argue that this behavior is the record of the progressive destabilization of garnet in a decompressive path from HP-HT conditions. The increase observed in the ratio is consistent with a progressive destabilization of plagioclase, which is the main europium reservoir in rocks (Barth and Wooden, 2010; Castiñeiras et al., 2011).
The sharp decrease observed in the Yb∕Gd ratio from 420 to 380 Ma is probably related to a new event of garnet growth (Rubatto et al., 2006; Stipska et al., 2016), i.e., the second HP-HT event. The evolution in the ratio suggests that this event took place under granulite facies conditions, as plagioclase was present to pump out all the available europium.
5.3 Monazite and titanite geochronology: age of the youngest metamorphism in the Sobrado antiform
The youngest zircon data recorded (380 ± 4 Ma) are consistent with the monazite concordia age (382 ± 1 Ma) in the migmatitic paragneiss of the middle tectonic slice. Besides, both monazite and irregular/subround zircons share their microtextural setting along the migmatitic fabric, pointing to a coeval character with partial melting at relatively high P. Furthermore, the chemical profiles observed in the monazite suggest simultaneous crystallization of this mineral with garnet (Rubatto, 2002; Rubatto et al., 2006; Mottram et al., 2014; Holder et al., 2015). Negative Eu anomalies indicate a preferential incorporation of europium to feldspars, in particular to K-feldspar, during melt crystallization (Buick et al., 2010; Rubatto et al., 2013). These characteristics are compatible with monazite crystallization in a single pulse (MSWD < 1) in the presence of garnet, supporting its metamorphic origin. This Middle Devonian age can be interpreted to represent the minimum age of the youngest metamorphic event in the Sobrado unit, which reached high-P granulite facies (Fernández-Suárez et al., 2007; Ordóñez Casado et al., 2001). It is suggested that monazite captures the onset of the exhumation process in the migmatitic paragneiss (Holder et al., 2015).
Titanite crystallization is syn-kinematic with the mylonitic fabric of the fine-grained amphibolites located at the base of the upper tectonic slice. The growth of titanite in amphibolitic conditions is supported by the umbrella-shaped REE patterns shown in Fig. 7b, typical of titanite coexisting with amphibole (Mulrooney and Rivers, 2005; Chambefort et al., 2013; Lesnov, 2013). A Late Devonian age (∼365 Ma) could be related to the onset of retrograde metamorphic conditions during the development of the shear zone and suggests a prolongated exhumation process reaching amphibolite facies. The Late Devonian age lies close to the Ar–Ar exhumation ages of the uppermost units in the Órdenes Complex as recorded in the Corredoiras detachment (376 ± 2.0 Ma in hornblende; Dallmeyer et al., 1997), or in the Ponte Carreira Detachment (371 ± 4.0 Ma in muscovite; Gómez Barreiro et al. 2006).
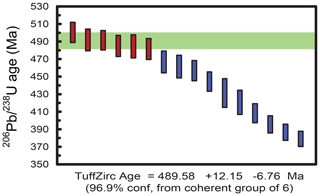
Figure 16Age of the onset of the oldest HP-HT metamorphic event obtained using the TuffZirc algorithm.
The U–Pb zircon age for the onset of the oldest metamorphic event was estimated using the TuffZirc method, developed by Ludwig and Mundil (2002), which calculates the median by choosing the largest set of concordant analyses that are statistically coherent. The best estimate obtained for this event is 489.58 (+12.15–6.76) Ma, obtained by pooling together only six of 16 analyses (Fig. 16). The 510–380 Ma zircon aliquot shows a clear correlation between its cathodoluminescence texture and its geochemistry and could be also related to shielded population of “metamorphic” zircon grains within garnets (Fig. 4e). The age recorded in the migmatitic paragneiss is thought to correspond to a metamorphic event, dated in the Early Ordovician (∼490 Ma) and is in very good agreement with upper high-P/high-T dates of equivalent units carried out during previous studies (Kuijper, 1979; Peucat et al., 1990; Fernández-Suárez et al., 2002, 2007). This age also coincides with those obtained from intermediate-P units, where large plutons were emplaced and there is a lack of later high-P/high-T metamorphism during the Devonian. The westernmost upper intermediate-P units of the Órdenes Complex underwent a granulite facies metamorphism dated between approximately 500 and 485 Ma, contemporaneous with the intrusion of massive gabbros and granodiorites related to Cambrian magmatic arc activity (Abati et al., 1999, 2003, 2007; Andonaegui et al., 2002, 2012, 2016; Castiñeiras et al., 2002, 2010). The granulite facies metamorphism is associated with heating produced by the intrusions, accompanied by a quick burial, almost coeval with igneous emplacement (Abati et al., 2003; Castiñeiras, 2005; Fernández-Suárez et al., 2007).
Clearly, the metamorphic event recorded in some zircons is pre-Variscan, and it is therefore independent of the high-P/high-T granulite facies metamorphism that occurred during the Early–Middle Devonian that has been identified in the underlying upper units, such as in Sobrado with 660–770 ∘C and 13–17 kbar (Arenas and Martínez Catalán, 2002) or 750–850 ∘C and 12–15 kbar (Benítez-Pérez, 2017). The pre-Variscan metamorphism was probably followed by a decompression stage, associated with partial melting (Fernández-Suárez et al., 2002). Later on, HP-HT Devonian metamorphism occurred, during which exhumation through an isothermal decompression led to partial melting in paragneiss and basic granulites (Fernández-Suárez et al., 2007). As the zircon composition and microstructural setting clearly suggest, the notable slope observed in the TuffZirc plot from 489 to 380 Ma (Fig. 16) is the result of these exhumation, burial and new exhumation processes accompanied by partial melting, in which the shielding role of garnet has been important.
This study provides new age constraints on the processes that have affected the Sobrado unit, part of the Órdenes Complex, and allows some correlation with events recognized in other parts of the allochthonous high-P/high-T complexes of NW Spain. Titanite, monazite and zircon dating together with REE analyses have been combined together in these rocks for the first time in order to carry out a geochronological investigation of the amphibolites and paragneiss.
According to the analyses, the youngest ages recorded by the metamorphic zircons are consistent with the concordia monazite age obtained from 76 analyses in the paragneiss. The microtextural setting of both metamorphic zircon and monazite along the HP-HT main foliation support that interpretation. Therefore, the Middle Devonian age (∼380 Ma) represents the minimum age of the last Sobrado metamorphic event under high-P granulite facies conditions and represents the first stages of the Variscan orogeny in this part of Spain. Dating of metamorphic titanite in the amphibolite yields a Late Devonian age (∼365 Ma) and is associated with very homogeneous REE patterns suggesting the prolongation of the exhumation process in the Sobrado unit, reaching amphibolite facies metamorphic conditions. In zircon, there is a strong relationship between their textures, as seen in cathodoluminescence images, REE patterns and 206Pb∕238U ages. Metamorphic zircon defines an Early Ordovician age (∼490 Ma) although it shows a large dispersion. This date is linked to the first pre-Variscan granulite facies metamorphism seen in the Sobrado unit under intermediate-P conditions, and it is interpreted to be related to the intrusion of basic and intermediate composition rocks, and coeval with burial in a magmatic arc context. Microstructural analysis of zircon, monazite and titanite provide complementary microtextural context to understand the origin of this population mixture. In situ dating should be conducted to confirm some textural relationships in the future.
The maximum depositional age of the Sobrado unit is suggested to be late Cambrian based on the age of the youngest inherited zircon (511 Ma). From the youngest set of inherited zircon, two ages can be obtained (546 and 526 Ma), pointing to the formation of a peri-Gondwanan magmatic arc. The protoliths of inherited zircon older than 1000 Ma from the Sobrado unit are found in other Spanish complexes and are thought to be related to sources mainly in the West African Craton.
The data are not publicly accessible.
JMBP, PC, JGB and JRMC contributed equally to the fieldwork, experimental process and elaboration of the manuscript. AKC contributed to U–Pb–REE acquisition and data reduction, and RH participated in the writing of the text and the geological interpretation.
The authors declare that they have no conflict of interest.
We kindly appreciate excellent reviews from Michele Zucali, Federico Rossetti, Puy Ayarza and an anonymous referee which contributed to improving the manuscript.
This research has been supported by the Spanish Ministry of Economy, Industry and Competitiveness (grant no. CGL2016-78560-P), the Spanish Ministry of Economy, Industry and Competitiveness (grant no. CGL2011-22728), the Spanish Ministry of Science and Innovation (grant no. IEDI-2016-00691) and the Spanish Ministry of Economy, Industry and Competitiveness (grant no. FPI 2013-2016 (BES-2012-059893)).
This paper was edited by Federico Rossetti and reviewed by Michele Zucali and one anonymous referee.
Abati, J., Dunning, G. R., Arenas, R., Díaz García, F., González Cuadra, P., Martínez Catalán, J. R., and Andonaegui, P.: Early Ordovician orogenic event in Galicia (NW Spain): Evidence from U-Pb ages in the uppermost unit of the Ordenes Complex, Earth Planet. Sc. Lett., 165, 213–228, https://doi.org/10.1016/S0012-821X(98)00268-4, 1999.
Abati, J., Arenas, R., Martinez Catalán, J. R., and Díaz García, F.: Anticlockwise P-T Path of Granulites from the Monte Castelo Gabbro (Ordenes Complex, NW Spain), J. Petrol., 44, 305–327, https://doi.org/10.1093/petrology/44.2.305, 2003.
Abati, J., Castiñeiras, P., Arenas, R., Fernández-Suárez, J., Barreiro, J. G., and Wooden, J. L.: Using SHRIMP zircon dating to unravel tectonothermal events in arc environments. The early Palaeozoic arc of NW Iberia revisited, Terra Nova, 19, 432–439, https://doi.org/10.1111/j.1365-3121.2007.00768.x, 2007.
Abati, J., Gerdes, A., Suárez, J. F., Arenas, R., Whitehouse, M. J., and Fernández, R. D.: Magmatism and early-Variscan continental subduction in the northern Gondwana margin recorded in zircons from the basal units of Galicia, NW Spain, Bull. Geol. Soc. Am., 122, 219–235, https://doi.org/10.1130/B26572.1, 2010.
Aerden, D. G. A. M.: Correlating deformation in Variscan NW-Iberia using porphyroblasts; implications for the Ibero-Armorican Arc, J. Struct. Geol., 26, 177–196, https://doi.org/10.1016/S0191-8141(03)00070-1, 2004.
Albert, R., Arenas, R., Gerdes, A., Sánchez-Martínez, S., Fernández-Suárez, J., and Fuenlabrada, J. M.: Provenance of the Variscan Upper Allochthon (Cabo Ortegal Complex, NW Iberian Massif), Gondwana Res., 28, 1434–1448, https://doi.org/10.1016/j.gr.2014.10.016, 2015.
Aleinikoff, J. N., Schenck, W. S., Plank, M. O., Srogi, L. A., Fanning, C. M., Kamo, S. L., and Bosbyshell, H.: Deciphering igneous and metamorphic events in high-grade rocks of the Wilmington complex, Delaware: Morphology, cathodoluminescence and backscattered electron zoning, and SHRIMP U-Pb geochronology of zircon and monazite, Bull. Geol. Soc. Am., 118, 39–64, https://doi.org/10.1130/B25659.1, 2006.
Aleinikoff, J. N., Wintsch, R. P., Tollo, R. P., Unruh, D. M., Fanning, C. M., and Schmitz, M. D.: Ages and origins of rocks of the Killingworth dome, south-central Connecticut: Implications for the tectonic evolution of southern New England, Am. J. Sci., 307, 63–118, https://doi.org/10.2475/01.2007.04, 2007.
Alvarez-Valero, A. M., Gómez-Barreiro, J., Alampi, A., Castineiras, P., and Martinez Catalán, J. R.: Local isobaric heating above an extensional detachment in the middle crust of a Variscan allochthonous terrane (Ordenes complex, NW Spain), Lithosphere-US, 6, 409–418, https://doi.org/10.1130/L369.1, 2014.
Andonaegui, P., Castiñeiras, P., González Cuadra, P., Arenas, R., Sánchez Martínez, S., Abati, J., Díaz García, F., and Martínez Catalán, J. R.: The Corredoiras orthogneiss (NW Iberian Massif): Geochemistry and geochronology of the Paleozoic magmatic suite developed in a peri-Gondwanan arc, Lithos, 128–131, 84–99, https://doi.org/10.1016/j.lithos.2011.11.005, 2012.
Andonaegui, P., González Del Tánago, J., Arenas, R., Abati, J., Martínez Catalán, J. R., Peinado, M., and Díaz García, F.: Tectonic setting of the Monte Castelo gabbro (Ordenes Complex, northwestern Iberian Massif): Evidence for an arc-related terrane in the hanging wall to the Variscan suture, Geol. S. Am. S., 364, 37–56, https://doi.org/10.1130/0-8137-2364-7.37, 2002.
Andonaegui, P., Sánchez-Martínez, S., Castiñeiras, P., Abati, J., and Arenas, R.: Reconstructing subduction polarity through the geochemistry of mafic rocks in a Cambrian magmatic arc along the Gondwana margin (Órdenes Complex, NW Iberian Massif), Int. J. Earth Sci., 105, 713–725, https://doi.org/10.1007/s00531-015-1195-x, 2016.
Arenas, R., and Martínez Catalán, J. L.: Prograde development of corona textures in metagabbros of the Sobrado unit (Órdenes Complex, northwestern Iberian Massif): Variscan-Appalachian dynamics: The building of the late Paleozoic basement, Special Papers Geological Society of America, 364, 73–88, https://doi.org/10.1130/0-8137-2364-7.73, 2002.
Bacon, C. R., Vazquez, J. A., and Wooden, J. L.: Peninsular terrane basement ages recorded by Paleozoic and Paleoproterozoic zircon in gabbro xenoliths and andesite from Redoubt volcano, Alaska, Bull. Geol. Soc. Am., 124, 24–34, https://doi.org/10.1130/B30439.1, 2012.
Ballèvre, M., Martínez Catalán J. R., López-Carmona, A., Pitra, P., Abati, J., Díez Fernández, R., Ducassou, C., Arenas, R., Bosse, V., Castiñeiras, P., Fernández-Suárez, J., Gómez Barreiro, J., Paquette, J.-L., Peucat, J.-J., Poujol, M., Ruffet, G., and Sánchez Martínez, S.: Correlation of the nappe stack in the Ibero-Armorican arc across the Bay of Biscay: a joint French–Spanish project, Geological Society, London, Special Publications, 405, 77–113, https://doi.org/10.1144/SP405.13, 2014.
Barth, A. P. and Wooden, J. L.: Coupled elemental and isotopic analyses of polygenetic zircons from granitic rocks by ion microprobe, with implications for melt evolution and the sources of granitic magmas, Chem. Geol., 277, 149–159, https://doi.org/10.1016/j.chemgeo.2010.07.017, 2010.
Belousova, E. A., Griffin, W. L., O'Reilly, S. Y., and Fisher, N. I.: Igneous zircon: trace element composition as an indicator of source rock type, Contrib. Mineral. Petr., 143, 602–622, https://doi.org/10.1007/s00410-002-0364-7, 2002.
Benítez-Pérez, J. M.: Quantitative study of texture in tectonites by diffraction. Contribution to seismic anisotropy and orogenic rheology, PhD. Thesis, University of Salamanca, Spain, 2017.
Buick, I. S., Clark, C., Rubatto, D., Hermann, J., Pandit, M., and Hand, M.: Constraints on the Proterozoic evolution of the Aravalli-Delhi Orogenic belt (NW India) from monazite geochronology and mineral trace element geochemistry, Lithos, 120, 511–528, https://doi.org/10.1016/j.lithos.2010.09.011, 2010.
Castiñeiras, P., Andonaegui, P., Arenas, R., and Martinez Catalán, J.: Descripción y resultados preliminares del plutón compuesto de San Xiao, Complejo de Cabo Ortegal (noroeste del Macizo Ibérico), Geogaceta, 32, 111–114, 2002.
Castiñeiras, P.: Origen y evolución tectonotermal de las unidades de O Pino y Cariño (Complejos Alóctonos de Galicia), Laboratorio Xeolóxico de Laxe, Serie Nova Terra, 28, A Coruña., 2005.
Castiñeiras, P., García, F. D., and Gómez-Barreiro, J.: REE-assisted U-Pb zircon age (SHRIMP) of an anatectic granodiorite: Constraints on the evolution of the A Silva granodiorite, Iberian allochthonous complexes, Lithos, 116, 153–166, https://doi.org/10.1016/j.lithos.2010.01.013, 2010.
Castiñeiras, P., Navidad, M., Casas, J. M., Liesa, M., and Carreras, J.: Petrogenesis of Ordovician Magmatism in the Pyrenees (Albera and Canigó Massifs) Determined on the Basis of Zircon Minor and Trace Element Composition, J. Geol., 119, 521–534, https://doi.org/10.1086/660889, 2011.
Chen, R. X., Zheng, Y. F., and Xie, L.: Metamorphic growth and recrystallization of zircon: Distinction by simultaneous in-situ analyses of trace elements, U-Th-Pb and Lu-Hf isotopes in zircons from eclogite-facies rocks in the Sulu orogen, Lithos, 114, 132–154, https://doi.org/10.1016/j.lithos.2009.08.006, 2010.
Cheng, H., King, R. L., Nakamura, E., Vervoort, J. D., Zheng, Y. F., Ota, T., Wu, Y. B., Kobayashi, K., and Zhou, Z. Y.: Transitional time of oceanic to continental subduction in the Dabie orogen: Constraints from U-Pb, Lu-Hf, Sm-Nd and Ar-Ar multichronometric dating, Lithos, 110, 327–342. https://doi.org/10.1016/j.lithos.2009.01.013, 2009.
Cherniak, D. J.: Zr diffusion in titanite, Contrib. Mineral. Petr., 152, 639–647, https://doi.org/10.1007/s00410-006-0133-0, 2006.
Corfu, F., Hanchar, J. M., Hoskin, P. W. O., and Kinny, P. D.: Atlas of Zircon Textures, in: Zircon. Reviews in Mineralogy and Geochemistry, edited by: Hanchar, J. M. and Hoskin, P. W. O. Mineralogical Society of America, 468–500, 2003.
Corrie, S. L. and Kohn, M. J.: Trace-element distributions in silicates during prograde metamorphic reactions?: implications for monazite formation, Journal of Metamorphic Geology, 26, 451–464, https://doi.org/10.1111/j.1525-1314.2008.00769.x, 2008.
Dallmeyer, R. D., Martínez Catalán, J. R., Arenas, R., Gil Ibarguchi, J. I., Gutiérrez-Alonso, G., Farias, P., Bastida, F., and Aller, J.: Diachronous Variscan tectonothermal activity in the NW Iberian Massif: Evidence from 40Ar/39Ar dating of regional fabrics, Tectonophysics, 277, 307–337, https://doi.org/10.1016/S0040-1951(97)00035-8, 1997.
Dallmeyer, R. D., Ribeiro, A., and Marques, F.: Polyphase Variscan emplacement of exotic terranes (Morais and Bragança Massifs) onto Iberian successions: Evidence from 40Ar/39Ar mineral ages, Lithos, 27, 133–144, https://doi.org/10.1016/0024-4937(91)90025-G, 1991.
de Pablo Maciá, J. G. and Martínez Catalán, J. R.: Estructura, petrología y evolución de la región de Sobrado de los Monjes (La Coruña), Cad. Lab. Xeol. Laxe., 7, 103–124, 1984.
DeWolf, C. P., Belshaw, N., and O'Nions, R. K.: A metamorphic history from micron-scale 207Pb/206Pb chronometry of Archean monazite, Earth Planet. Sc. Lett., 120, 207–220, 1993.
Dias da Silva, I., Valverde-Vaquero, P., González-Clavijo, E., Díez-Montes, A., and y Martínez Catalán, J. R.: Structural and stratigraphical significance of U–Pb ages from the Mora and Saldanha volcanic complexes (NE Portugal, Iberian Variscides), in: Schulmann, K., Martínez Catalán, J. R., Lardeaux, J. M., Janousek, V., and Oggiano, G.: The Variscan Orogeny: Extent, Timescale, the Formation of the European Crust, Geological Society, London, Special Publications, 405, https://doi.org/10.1144/SP405.3, 2014.
Díaz García, F., Martínez Catalán, J. R., Arenas, R., and González Cuadra, P.: Structural and kinematic analysis of the Corredoiras detachment: evidence for early Variscan synconvergent extension in the Ordenes Complex, NW Spain, Int. J. Earth Sci., 88, 337–351, 1999.
Díez Fernández, R., Catalán, J. R. M., Gerdes, A., Abati, J., Arenas, R., and Fernández-Suárez, J.: U–Pb ages of detrital zircons from the Basal allochthonous units of NW Iberia: Provenance and paleoposition on the northern margin of Gondwana during the Neoproterozoic and Paleozoic. Gondwana Res., 18, 385–399, https://doi.org/10.1016/j.gr.2009.12.006, 2010.
Díez Fernández, R., Martínez Catalán, J. R., Arenas, R., Abati, J., Gerdes, A., and Fernández-Suárez, J.: U–Pb detrital zircon analysis of the lower allochthon of NW Iberia: age constraints, provenance and links with the Variscan mobile belt and Gondwanan cratons, J. Geol. Soc. London, 169, 655–665, https://doi.org/10.1144/jgs2011-146, 2012.
Egal, E., Thiéblemont, D., Lahondère, D., Guerrot, C., Costea, C. A., Iliescu, D., Delor, C., Goujou, J. C., Lafon, J. M., Tegyey, M., Diaby, S., and Kolié, P.: Late Eburnean granitization and tectonics along the western and northwestern margin of the Archean Kenema-Man domain (Guinea, West African Craton), Precambrian Res., 117, 57–84, https://doi.org/10.1016/S0301-9268(02)00060-8, 2002.
Ennih, N. and Liegeois, J.-P.: The boundaries of the West African craton, with special reference to the basement of the Moroccan metacratonic Anti-Atlas belt, The Boundaries of the West African Craton, 1–17, https://doi.org/10.1144/SP297.1, 2008.
Farias, P., Gallastegui, G., González Lodeiro, F., Marquínez, J., Martín Parra, L. M., Martínez Catalán, J. R., de Pablo Maciá, J. G., and Rodríguez Fernández, L. R.: Aportaciones al conocimiento de la litoestratigrafía y estructura de Galicia Central. Memórias da Faculdade de Ciências, Universidade do Porto 1, 411–431, 1987.
Fernández-Suárez, J., Arenas, R., Abiati, J., Martinez Catalán, J. R., Whitehouse, M., and Jeffries, T.: U-Pb chronometry of polymetamorphic high-pressure granulites: An example from the allochthonous terranes of the NW Iberian Variscan belt, Geol. Soc. Am. Mem., 200, 469–488, https://doi.org/10.1130/2007.1200(24), 2007.
Fernández-Suárez, J., Corfu, F., Arenas, R., Marcos, A., Martínez Catalán, J. R., Díaz García, F., Abati, J., and Fernández, F. J.: U-Pb evidence for a polyorogenic evolution of the HP-HT units of the NW Iberian Massif, Contrib. Mineral. Petr., 143, 236–253, https://doi.org/10.1007/s00410-001-0337-2, 2002.
Fernández-Suárez, J., Garcia, F. D., Jeffries, T. E., and Arenas, R.: Constraints on the provenance of the uppermost allochthonous terrane of the NW Iberian Massif: inferences from detrital zircon U-Pb ages, Terra Nova, 15, 138–144, https://doi.org/10.1046/j.1365-3121.2003.00479.x, 2003.
Franz, G. and Spear, F. S.: Aluminous Titanite (sphene) from the Eclogite Zone, south-central Tauern window, Austria, Chem. Geol., 50, 33–46, https://doi.org/10.1016/0009-2541(85)90110-X, 1985.
Frost, B. R., Chamberlain, K. R., and Schumacher, J.C.: Sphene (titanite): phase relations and role as a geochronometer, Chem. Geol., 172, 131–148, https://doi.org/10.1016/S0009-2541(00)00240-0, 2000.
Fuenlabrada, J., Arenas, R., Sánchez-Martínez, S., Díaz García, F., and Castiñeiras, P.: A peri-Gondwanan arc in NW Iberia I: Isotopic and geochemical constraints on the origin of the arc-A sedimentary approach, Gondwana Res., 17, 338–351, https://doi.org/10.1016/j.gr.2009.09.007, 2010.
Gagnevin, D. and Daly, J. S.: Zircon texture and chemical composition as a guide to magmatic processes and mixing in a granitic environment and coeval volcanic system, https://doi.org/10.1007/s00410-009-0443-0, 2010.
Gómez Barreiro, J., Wijbrans, J. R., Castiñeiras, P., Martínez Catalán, J. R., Arenas, R., Díaz García, F., and Abati, J.: 40Ar/39Ar laserprobe dating of mylonitic fabrics in a polyorogenic terrane of NW Iberia, J. Geol. Soc. London, 163, 61–73, https://doi.org/10.1144/0016-764905-012, 2006.
Gómez-Barreiro, J., Martínez Catalán, J. R., Arenas, R., Castiñeiras, P., Abati, J., Díaz García, F., and Wijbrans, J. R.: Tectonic evolution of the upper allochthon of the Órdenes complex (northwestern Iberian Massif): Structural constraints to a polyorogenic peri-Gondwanan terrane, Geol. S. Am., 423, 315–332, https://doi.org/10.1130/2007.2423(15), 2007.
Goncalves, P., Williams, M. L., and Jercinovic, M. J.: Electron-microprobe age mapping of monazite, Am. Mineral., 90, 578–585, https://doi.org/10.2138/am.2005.1399, 2005.
Grimes, C. B., Wooden, J. L., Cheadle, M. J., and John, B. E.: “Fingerprinting” tectono-magmatic provenance using trace elements in igneous zircon, Contrib. Mineral. Petr., 170, 1–26, https://doi.org/10.1007/s00410-015-1199-3, 2015.
Hacker, B. R., Kylander-clark, A. R. C., Holder, R., Andersen, T. B., Peterman, E. M., Walsh, E. O., and Munnikhuis, J. K.: Monazite response to ultrahigh-pressure subduction from U-Pb dating by laser ablation split stream, Chem. Geol., 409, 28–41, https://doi.org/10.1016/j.chemgeo.2015.05.008, 2015.
Hanchar, J. M. and Van Westrenen, W.: Rare earth element behavior in zircon-melt systems, Elements, 3, 37–42, https://doi.org/10.2113/gselements.3.1.37, 2007.
Harlov, D., Tropper, P., Seifert, W., Nijland, T., and Förster, H.-J.: Formation of Al-rich titanite (CaTiSiO4O – CaAlSiO4OH) reaction rims on ilmenite in metamorphic rocks as a function of fH2O and fO2, Lithos, 88, 72–84, https://doi.org/10.1016/j.lithos.2005.08.005, 2006.
Hawkins, D. P. and Bowring, S. A.: U-Pb systematics of monazite and xenotime?: case studies from the Paleoproterozoic of the Grand Canyon, Arizona, 87–103, 1997.
Hermann, J. and Rubatto, D.: Relating zircon and monazite domains to garnet growth zones?: Age and duration of granulite facies metamorphism in the Val Malenco lower crust, Journal of Metamorphic, 21, 833–852, https://doi.org/10.1046/j.1525-1314.2003.00484.x, 2003.
Hokada, T. and Harley, S. L.: Zircon growth in UHT leucosome: constraints from zircon-garnet rare earth elements (REE) relations in Napier Complex, East Antarctica, J. Miner. Petrol. Sci., 99, 180–190, https://doi.org/10.2465/jmps.99.180, 2004.
Holder, R. M., Hacker, B. R., Kylander-Clark, A. R. C., and Cottle, J. M.: Monazite trace-element and isotopic signatures of (ultra) high-pressure metamorphism?: Examples from the Western Gneiss Region, Norway, Chem. Geol., 409, 99–111, https://doi.org/10.1016/j.chemgeo.2015.04.021, 2015.
Horstwood, M. S. A., Foster, G. L., Parrish, R. R., Noble, S. R., and Nowell, G. M.: Common-Pb corrected in situ U–Pb accessory mineral geochronology by LA-MC-ICP-MS, J. Anal. At. Spectrom, 18, 837–846, https://doi.org/10.1039/b304365g, 2003.
Hoskin, P. W. O.: Trace-element composition of hydrothermal zircon and the alteration of Hadean zircon from the Jack Hills, Australia, Geochim. Comochim. Ac., 69, 637–648, https://doi.org/10.1016/j.gca.2004.07.006, 2005.
Hoskin, P. W. O. and Ireland, T. R.: Rare earth element chemistry of zircon and its use as a provenance indicator, Geology, 28, 627–630, https://doi.org/10.1130/0091-7613(2000)28<627:REECOZ>2.0.CO;2, 2000.
Hoskin, P. W. O. and Schaltegger, U.: The Composition of Zircon and Igneous and Metamorphic Petrogenesis, in: Hanchar, J. M. and Hoskin, P. W. O., Zircon, Reviews in Mineralogy and Geochemistry, Mineralogical Society of America, 27–62, 2003.
Jackson, S. E., Pearson, N. J., Griffin, W. L., and Belousova, E. A.: The application of laser ablation-inductively coupled plasma-mass spectrometry to in situ U-Pb zircon geochronology, Chem. Geol., 211, 47–69, https://doi.org/10.1016/j.chemgeo.2004.06.017, 2004.
Ji, S. and Martignole, J.: Ductility of garnet as an indicator of extremely high temperature deformation, J. Struct. Geol., 16, 985–996, 1994.
Kleinschrodt, R. and Duyster, J.: HT-deformation of garnet: an EBSD study on granulites from Sri Lanka, India and the Ivrea Zone, J. Struct. Geol., 24, 1829–1844, 2002.
Kohn, M. J. and Malloy, M. A.: Formation of monazite via prograde metamorphic reactions among common silicates?: Implications for age determinations, Geochim. Comochim. Ac., 68, 101–113, https://doi.org/10.1016/S0016-7037(03)00258-8, 2004.
Košler, J., Tubrett, M. N., and Sylvester, P. J.: Application of laser ablation ICP-MS to U-Th-Pb dating of monazite, Geostandard Newslett., 25, 375–386, https://doi.org/10.1111/j.1751-908X.2001.tb00612.x, 2001.
Kuijper, R. P.: U-Pb systematic and petrogenetic evolution of infracrustal rocks in the Paleozoic basement of Western Galicia, NW Iberia, WO Laboratory of Isotope Geology, Amsterdam, 1979.
Kylander-Clark, A. R. C., Hacker, B. R., and Cottle, J. M.: Laser-ablation split-stream ICP petrochronology. Chem. Geol., 345, 99–112, https://doi.org/10.1016/j.chemgeo.2013.02.019, 2013.
Lesnov, F. P.: Consistent patterns of rare earth element distribution in accessory minerals from rocks of mafic-ultramafic complexes, Cent. Eur. J. Geosci., 5, 112–173, https://doi.org/10.2478/s13533-012-0121-z, 2013.
Ludwig, K. R.: On the Treatment of Concordant Uranium-Lead Ages, Geochim. Comochim. Ac., 62, 665–676, https://doi.org/10.1016/S0016-7037(98)00059-3, 1998.
Ludwig, K. R.: User's Manual for ISOPLOT version 3.75, A Geochronological Toolkit for Microsoft Excel, Berkeley Geochronology Center, 2012.
Ludwig, K. R. and Mundil, R.: Extracting reliable U–Pb ages and errors from complex populations of zircons from Phanerozoic tuffs, Geochim. Comochim. Ac., 66, 463, 2002.
Martínez Catalán, J. R., Arenas, R., Díaz García, F., and Abati, J.: Allochthonous Units in the Variscan Belt of NW Iberia: Terranes and Accretionary History, in: Basement Tectonics 13, edited by: Sinha, A. K., Proceedings of the Thirteenth International Conference on Basement Tectonics Held in Blacksburg, Virginia, USA, June 1997, Springer Netherlands, Dordrecht, 65–84, https://doi.org/10.1007/978-94-011-4800-9_5, 1999.
Martínez Catalán, J. R., Fernández-Suárez, J., Jenner, G. A., Belousova, E., and Díez Montes, A.: Provenance constraints from detrital zircon U–Pb ages in the NW Iberian Massif: implications for Paleozoic plate configuration and Variscan evolution, J. Geol. Soc. London, 161, 461–473, https://doi.org/10.1144/0016-764903-054, 2004,
Martínez Catalán, J. R., Arenas, R., Abati, J., Sánchez Martínez, S., Díaz García, F., Fernaández Suaárez, J., González Cuadra, P., Castiñeiras, P., Gómez Barreiro, J., Díez Montes, A., González Clavijo, E., Rubio Pascual, F. J., Andonaegui, P., Jeffries, T. E., Alcock, J. E., Diíez Fernández, R., and López Carmona, A.: A rootless suture and the loss of the roots of a mountain chain: The Variscan belt of NW Iberia, C. R. Geosci., 341, 114–126, https://doi.org/10.1016/j.crte.2008.11.004, 2009.
Martínez Catalán, J. R.: Are the oroclines of the Variscan belt related to late Variscan strike-slip tectonics?, Terra Nova, 23, 241–247, https://doi.org/10.1111/j.1365-3121.2011.01005.x, 2011.
Martínez Catalán, J. R.: The Central Iberian arc, an orocline centered in the Iberian Massif and some implications for the Variscan belt, Int. J. Earth Sci., 101, 1299–1314, https://doi.org/10.1007/s00531-011-0715-6, 2012.
McDonough, W. F. and Sun, S. S.: The composition of the Earth, Chem. Geol., 120, 223–253, https://doi.org/10.1016/0009-2541(94)00140-4, 1995.
Möller, A., O'Brien, P. J., Kennedy, A., and Kröner, A.: Polyphase zircon in ultrahigh-temperature granulites (Rogaland, SW Norway): Constraints for Pb diffusion in zircon, J. Meetamorph. Geol., 20, 727–740, https://doi.org/10.1046/j.1525-1314.2002.00400.x, 2002.
Mottram, C. M., Warren, C. J., Regis, D., Roberts, N. M. W., Harris, N. B. W., Argles, T. W., and Parrish, R. R.: Developing an inverted barrovian sequence; insights from monazite petrochronology, Earth Planet. Sc. Lett., 403, 418–431, https://doi.org/10.1016/j.epsl.2014.07.006, 2014.
Mulrooney, D. and Rivers, T.: Redistribution of the rare-earth elements among coexisting minerals in metamafic rocks across the epidote-out isograd: An example from the St. Anthony Complex, northern Newfoundland, Canada, Can. Mineral., 43, 263–294, https://doi.org/10.2113/gscanmin.43.1.263, 2005.
Nasdala, L., Zhang, M., Kempe, U., Panczer, G., Gaft, M., Andrut, M., and Plötze, M.: Spectroscopic methods applied to zircon, in: Zircon. Reviews in Mineralogy and Geochemistry, edited by: Hanchar, J. M. and Hoskin, P. W. O., Mineral. Soc. Am., 427–467, 2003.
Ordóñez Casado, B.: Geochronological studies of the pre-Mesozoic basement of the Iberian Massif?: the Ossa Morena zone and the Allochthonous Complexes within the Central Iberian zone, PhD thesis, Swiss Federal Institute of Technology, Zürich, Switzerland, 235 pp., https://doi.org/10.3929/ethz-a-002017279, 1998.
Ordóñez Casado, B., Gebauer, D., Schäfer, H. J., Ibarguchi, J. I. G., and Peucat, J. J.: A single Devonian subduction event for the HP/HT metamorphism of the Cabo Ortegal complex within the Iberian Massif, Tectonophysics, 332, 359–385, https://doi.org/10.1016/S0040-1951(00)00210-9, 2001.
Palin, R. M., Searle, M. P., Waters, D. J., Parrish, R. R., Roberts, N. M. W., Horstwood, M. S. A., Yeh, M. W., Chung, S. L., and Anh, T. T.: A geochronological and petrological study of anatectic paragneiss and associated granite dykes from the Day Nui Con Voi metamorphic core complex, North Vietnam: Constraints on the timing of metamorphism within the Red River shear zone, J. Meetamorph. Geol., 31, 359–387, https://doi.org/10.1111/jmg.12025, 2013.
Passchier, C. W. and Trouw, R. A. J.: Microtectonics, Springer Science & Business Media, Berlin, Heidelberg, 2005.
Paton, C., Hellstrom, J., Paul, B., Woodhead, J., and Hergt, J.: Iolite: Freeware for the visualisation and processing of mass spectrometric data, J. Anal. Atom. Spectrom., 26, 2508, https://doi.org/10.1039/c1ja10172b, 2011.
Peters, T. J., Ayers, J. C., Gao, S., and Liu, X. M.: The origin and response of zircon in eclogite to metamorphism during the multi-stage evolution of the Huwan Shear Zone, China: Insights from Lu-Hf and U-Pb isotopic and trace element geochemistry, Gondwana Res., 23, 726–747, https://doi.org/10.1016/j.gr.2012.05.008, 2013.
Peucat, J. J., Bernard Griffiths, J., Ibarguchi, J. I. G., Dallmeyer, R. D., Menot, R. P., Cornichet, J., and Ponce de León, M. I.: Geochemical and Geochronological Cross-Section of the Deep Variscan Crust – the Cabo-Ortegal High-Pressure Nappe (Northwestern Spain), Tectonophysics, 177, 263–292, 1990.
Peucat, J. J., Capdevila, R., Drareni, A., Mahdjoub, Y., and Kahoui, M.: The Eglab massif in the West African Craton (Algeria), an original segment of the Eburnean orogenic belt: Petrology, geochemistry and geochronology, Precambrian Res., 136, 309–352, https://doi.org/10.1016/j.precamres.2004.12.002, 2005.
Potrel, A., Peucat, J. J., and Fanning, C. M.: Archean crustal evolution of the West African Craton: example of the Amsaga Area (Reguibat Rise). U-Pb and Sm-Nd evidence for crustal growth and recycling, Precambrian Res., 90, 107–117, https://doi.org/10.1016/S0301-9268(98)00044-8, 1998.
Ribeiro, A., Pereira, E., and Dias, R.: Central-Iberian Zone. Allochthonous Sequences. Structure in the Northwest of the Iberian Peninsula, in: Pre-Mesozoic Geology of Iberia, edited by: Dallmeyer, R. D. and y Martínez García, E., Springer, Berlin, 220–236, 1990.
Rubatto, D.: Zircon trace element geochemistry?: distribution coefficients and the link between U-Pb ages and metamorphism Zircon trace element geochemistry?: partitioning with garnet and the link between U-Pb ages and metamorphism, Chem. Geol., 184, 123–138, 2002.
Rubatto, D., and Hermann, J.: Exhumation as fast as subduction?, Geology, 29, 3–6, 2001.
Rubatto, D., Hermann, R. G., and Buick, I. S.: Temperature and Bulk Composition Control on the Growth of Monazite and Zircon During Low-pressure Anatexis (Mount Stafford , Central Australia), J. Petrol., 47, 1973–1996, https://doi.org/10.1093/petrology/egl033, 2006.
Rubatto, D., Hermann, J., Berger, A., and Engi, M.: Protracted fluid-induced melting during Barrovian metamorphism in the Central Alps, Contrib. Mineral. Petr., 158, 703–722, https://doi.org/10.1007/s00410-009-0406-5, 2009.
Rubatto, D., Chakraborty, S., and Dasgupta, S.: Timescales of crustal melting in the Higher Himalayan Crystallines (Sikkim, Eastern Himalaya) inferred from trace element-constrained monazite and zircon chronology, Contrib. Mineral. Petr., 165, 349–372, https://doi.org/10.1007/s00410-012-0812-y, 2013.
Santos Zalduegui, J. F., Schärer, U., and Gil Ibarguchi, J. I.: Isotope constraints on the age and origin of magmatism and metamorphism in the Malpica-Tuy allochthon, Galicia, NW Spain. Chem. Geol., 121, 91–103, https://doi.org/10.1016/0009-2541(94)00123-P, 1995.
Santos Zalduegui, J. F., Schärer, U., Gil Ibarguchi, J. I., and Girardeau, J.: Origin and evolution of the Paleozoic Cabo Ortegal ultramafic-mafic complex (NW Spain): U-Pb, Rb-Sr and Pb-Pb isotope data, Chem. Geol., 129, 281–304, https://doi.org/10.1016/0009-2541(95)00144-1, 1996.
Santos Zalduegui, J. F., Schärer, U., Gil Ibarguchi, J. I., and Girardeau, J.: Genesis of Pyroxenite-rich Peridotite at Cabo Ortegal (NW Spain): Geochemical and Pb-Sr-Nd Isotope Data, J. Petrol., 43, 17–43, https://doi.org/10.1093/petrology/43.1.17, 2002.
Schofield, D. I., Horstwood, M. S. A., Pitfield, P. E. J., Gillespie, M., Darbyshire, F., O'Connor, E. A., and Abdouloye, T. B.: U-Pb dating and Sm-Nd isotopic analysis of granitic rocks from the Tiris Complex: New constaints on key events in the evolution of the Reguibat Shield, Mauritania, Precambrian Res., 204–205, 1–11, https://doi.org/10.1016/j.precamres.2011.12.008, 2012.
Spear, F. S.: An experimental study of hornblende stability and compositional variability in amphibolite, Am. J. Sci., 281, 697–734, https://doi.org/10.2475/ajs.281.6.697, 1981.
Spear, F. S. and Pyle, J. M.: Apatite , Monazite , and Xenotime in Metamorphic Rocks, in: Phosphates: Geochemical, Geobiological, and Materials Importance edited by: Kohn, M. J., Rakovan, J., and Hughes, J. M., Rev. Mineral. Geochem., 48, 293–335, https://doi.org/10.2138/rmg.2002.48.7, 2002.
Spencer, K. J., Hacker, B. R., Kylander-Clark, A. R. C., Andersen, T. B., Cottle, J. M., Stearns, M. A., Poletti, J. E., and Seward, G. G. E.: Campaign-style titanite U – Pb dating by laser-ablation ICP?: Implications for crustal flow, phase transformations and titanite closure, Chem. Geol., 341, 84–101, https://doi.org/10.1016/j.chemgeo.2012.11.012, 2013.
Stearns, M. A., Hacker, B. R., Ratschbacjer, L., Rutte, D., and Kylander-Clark, A. R. C.: Titanite petrochronology of the Pamir gneiss domes: Implications for middle to deep crust exhumation and titanite closure to Pb and Zr diffusion, Tectonics, 34, 784–802, https://doi.org/10.1002/2014TC003774, 2015.
Stearns, M. A., Cottle, J. M., Hacker, B. R., and Kylander-Clark, A. R. C.: Extracting thermal histories from the near-rim zoning in titanite using coupled U-Pb and trace-element depth profiles by single-shot laser-ablation split stream (SS-LASS) ICP-MS, Chem. Geol., 422, 13–24, https://doi.org/10.1016/j.chemgeo.2015.12.011, 2016.
Stipska, P., Powell, R., Hacker, B. R., and Holder, R.: Uncoupled U∕Pb and REE response in zircon during the transformation of eclogite to mafic and intermediate granulite (Blansk y les, Bohemian Massif), J. Metamorph. Geol., 34, 551–572, https://doi.org/10.1111/jmg.12193, 2016.
Storey, C. D., Smith, M. P., and Jeffries, T. E.: In situ LA-ICP-MS U – Pb dating of metavolcanics of Norrbotten, Sweden?: Records of extended geological histories in complex titanite grains, Chem. Geol., 240, 163–181, https://doi.org/10.1016/j.chemgeo.2007.02.004, 2007.
Stübner, K., Grujic, D., Parrish, R. R., Roberts, N. M. W., Kronz, A., Wooden, J., and Ahmad, T.: Lithos Monazite geochronology unravels the timing of crustal thickening in NW Himalaya, Lithos, 210–211, 111–128, https://doi.org/10.1016/j.lithos.2014.09.024, 2014.
Tera, F. and Wasserburg, G. J.: U-Th-Pb systematics in three Apollo 14 basalts and the problem of initial Pb in lunar rocks, Earth Planet. Sc. Lett., 14, 281–304, https://doi.org/10.1016/0012-821X(72)90128-8, 1972.
Terry, M. P. and Hamilton, M. A.: Monazite geochronology of UHP and HP metamorphism, deformation, and exhumation, Nordoyane, Western Gneiss Region, Norway, American Mineralogist, 85, 1651–1664, https://doi.org/10.2138/am-2000-11-1208, 2000.
Tomascak, P. B., Krogstad, E. J., and Walker, R. J.: U-Pb Monazite Geochronology of Granitic Rocks from Maine: Implications for Late Paleozoic Tectonics in the Northern Appalachians, J. Geol., 104, 185–195, 1996.
Verts, L. A. and Frost, C. D.: U-Pb sphene dating of metamorphism?: the importance of sphene growth in the contact aureole of the Red Mountain pluton, Laramie Mountains, Wyoming, Contrib. Mineral. Petr., 125, 186–199, https://doi.org/10.1007/s004100050215, 1996.
Watson, E. B. and Yan L.: A simple model for sector zoning in slowly grown crystals: implications for growth rate and lattice diffusion, with emphasis on accessory minerals in crustal rocks, Am. Mineral., 80, 1179–1187, 1995.
Whitehouse, M. J. and Platt, .J. P.: Dating high-grade metamorphism – constraints from rare-earth elements in zircon and garnet, Contrib. Mineral. Petr., 145, 61–74, https://doi.org/10.1007/s00410-002-0432-z, 2003.
Whitney, D. L. and Evans, B. W.: Abbreviations for names of rock-forming minerals, Am. Mineral., 95, 185–187, https://doi.org/10.2138/am.2010.3371, 2010.
Wieldenbeck, M., Allé, P., Corfú, F., Griffin, W. L., Meier, M., Oberli, F., von Quadt, A., Roddick, J. C., and Spiegel, W.: Three Natural Zircon Standards for U-Th-Pb, Lu-Hf, Trace Element and REE Analyses, Geostandard Newslett., 19, 1–23, https://doi.org/10.1111/j.1751-908X.1995.tb00147.x, 1995.
Williams, M. L., Jercinovic, M. J., and Hetherington, C. J.: Microprobe Monazite Geochronology: Understanding Geologic Processes by Integrating Composition and Chronology, Annu. Rev. Earth Pl. Sc., 35, 137–175, https://doi.org/10.1146/annurev.earth.35.031306.140228, 2007.
Zeck, H. P., Wingate, M. T. D., Pooley, G. D., Ugidos, and J. M.: A Sequence of Pan-African and Hercynian Events Recorded in Zircons from an Orthogneiss from the Hercynian Belt of Western Central Iberia – an Ion Microprobe U-Pb Study, Journal of Petrology, 45, 1613–1629, https://doi.org/10.1093/petrology/egh026, 2004.
Zeringue, J., Bowring, J. F., McLean, N. M., and Pastor, F.: Building Interactive Visualizations for Geochronological Data, AGU Fall Meeting Abstracts, 2014.
Zhu, X. K., O'Nions, R. K., Belshaw, N. S., and Gibb, A. J.: Significance of in situ SIMS chronometry of zoned monazite from the Lewisian granulites, northwest Scotland. Chem. Geol., 5, 35–53, https://doi.org/10.1016/S0009-2541(96)00103-9, 1997.