the Creative Commons Attribution 4.0 License.
the Creative Commons Attribution 4.0 License.
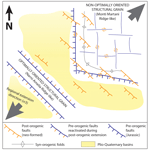
Reconciling post-orogenic faulting, paleostress evolution, and structural inheritance in the seismogenic northern Apennines (Italy): insights from the Monti Martani Fault System
Selina Bonini
Giulio Viola
Gianluca Vignaroli
Structural inheritance plays a significant role in the evolution of fault systems in different tectonic settings. Both positive reactivation of pre-orogenic extensional faults and negative reactivation of synorogenic reverse faults during orogenic cycles have been extensively studied and documented. By contrast, only a few studies have addressed the impact of structural inheritance in regions undergoing polyphasic tectonic histories. Here, we present the Monti Martani Fault System (MMFS) case study (northern Apennines, Italy) as a representative example of a seismically active region where one can investigate the role of inherited pre-orogenic structural features upon the post-orogenic tectonic evolution. We collected outcrop-scale fault slip data from there to constrain fault geometry and kinematics as inputs to paleostress analysis. Based on data from extensional faults that controlled the Plio-Quaternary evolution of the system, we propose that the MMFS does not consist of a ca. 30 km long, L-shaped single normal fault, as previously proposed in the literature, but is instead formed by a set of several shorter NW–SE-trending extensional faults arranged in an en echelon style. Paleostress analysis yielded three distinct extension directions during the Plio-Quaternary post-orogenic extension, oriented NE–SW, NNE–SSW, and NW–SE. We relate the first two directions to local orientation fluctuations of the regional stress field interacting with the moderately oblique inherited structural features and the latter direction to a short-lived orogen-parallel extensional event whose geodynamic causes remain unclear. We suggest that the NE–SW regional post-orogenic extension direction controls the orientation of most of the NW–SE-striking extensional faults, while the morphostructural trend of the Monti Martani Ridge and of its boundaries with the surrounding Plio-Quaternary Medio Tiberino and Terni basins is controlled by the strike of the ∼ N–S and ∼ E–W pre-orogenic (Jurassic) inherited structural grain, rather than by the orientation of the post-orogenic extension direction. We also discuss the implications of these observations on the seismotectonics of the MMFS. Our findings suggest that, in contrast to previous suggestions, the fault system cannot be classified as an active and capable structural feature.
- Article
(30652 KB) - Full-text XML
-
Supplement
(682 KB) - BibTeX
- EndNote
In areas affected by polyphasic deformation, structural inheritance exerts a significant influence upon stress distribution and deformation partitioning, thus controlling the balance between reactivation of pre-existing structures and rupture along newly formed faults (e.g., Viola et al., 2009, 2012; Autin et al., 2013; Brune, 2014; Scheiber and Viola, 2018; Wang et al., 2021; Hodge et al., 2024). While the role of synorogenic structures is well documented for active and fossil belts (e.g., Tavarnelli et al., 2004; Curzi et al., 2020; Del Ventisette et al., 2021; Viola et al., 2022; Tavani et al., 2023), it remains poorly understood how inherited pre-orogenic structural features are geometrically and kinematically linked to the final architecture of the post-orogenic fault system. While field-based structural studies have generally shown that the spatial distribution and orientation of post-orogenic faults tend to closely resemble those of the pre-orogenic inheritance (e.g., Mercuri et al., 2024), insights from modeling on extended regions suggest, on the other hand, that complex patterns of newly formed faults may also form in response to the imposed stress field, overprinting and reworking the original pre-orogenic structural framework (Autin et al., 2013; Brune, 2014; Wang et al., 2021). The way post-orogenic faults interact with the pre-orogenic setting has major implications for tectonically active regions, as fault geometry and its orientation to the background stress field impact upon earthquake recurrence (e.g., Cowie et al., 2012) and Coulomb stress transfer during seismic events (e.g., Mildon et al., 2017; Galderisi and Galli, 2020). Thus, it is particularly important to understand and constrain the role of structural inheritance in tectonically active regions that, as a result of complex and polyphasic tectonic histories, have become saturated with inherited structural features (e.g., Viola et al., 2009, 2012; Scheiber and Viola, 2018; Hodge et al., 2024). It is useful to be aware that the mechanical anisotropy defined by structural inheritance is not limited to pre-existing faults, particularly in polyphase tectonic regions. In such cases, it is more appropriate to refer to an “inherited structural grain”, which can be defined as the summation of, for example, stratigraphic, geometric, kinematic, and tectonic features that, as a whole, express the bulk anisotropy of the crustal block being later further deformed.
The Apennines are an excellent example of a tectonically active region recording a series of tectonic events, spanning from pre-orogenic rifting and synorogenic folding and thrusting to post-orogenic extension (e.g., Cosentino et al., 2010; Carminati et al., 2012; Cardello and Doglioni, 2015; Conti et al., 2020). Many studies have documented the inversion of inherited structures in the Apennines during both the synorogenic and post-orogenic phases (e.g., Butler et al., 2004; Tavarnelli et al., 2004; Scisciani, 2009; Calamita et al., 2011; Di Domenica et al., 2012; Pace and Calamita, 2014; Curzi et al., 2020; Del Ventisette et al., 2021; Viola et al., 2022; Tavani et al., 2023). Accordingly, the current seismicity related to the post-orogenic extensional regime in the inner part of the belt is likely affected by the pre-existing structural framework (e.g., Chiarabba and Amato, 2003; Buttinelli et al., 2018; Barchi et al., 2021). Recent studies have even documented polyphasic reactivations of pre-orogenic extensional faults during both positive and negative inversion (Mercuri et al., 2024).
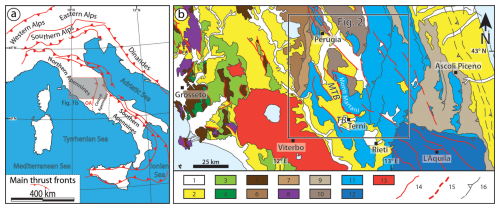
Figure 1(a) Schematic tectonic framework of the Italian peninsula with the location of the main thrust fronts and subdivision of the main domains of the Apennine belt (modified after Zuccari et al., 2022). OA: Olevano–Antrodoco tectonic line. (b) Tectonic map of the northern Apennines at the transition to the central Apennines, redrawn and modified after Conti et al. (2020). 1: Quaternary deposits; 2: Miocene–Pleistocene succession; 3: Ligurian units; 4: sub-Ligurian units (tectonic mélanges); 5: Tuscan Nappe; 6: Cervarola–Falterona unit; 7: Rentella unit; 8: Tuscan metamorphic units; 9: Umbria–Marche–Romagna unit (turbidite successions of “minor” and outer basins); 10: Umbria–Marche–Romagna unit (turbidite successions of the inner basins); 11: Umbria–Marche domain (Triassic–Miocene succession); 12: Lazio–Abruzzi carbonate units; 13: magmatic rocks; 14: normal faults; 15: Martani Fault System; 16: thrusts; MTB: Medio Tiberino Basin; TB: Terni Basin.
Such a complex structural framework and tectonic scenario is well exemplified by the Monti Martani Ridge (MMR) in the southernmost portion of the northern Apennines (Fig. 1b), where a Mesozoic–Cenozoic sedimentary succession crops out, forming an ∼ N–S structural ridge that is bounded by intermountain basins (Medio Tiberino and Terni basins) formed during post-orogenic extensional tectonics.
In this work, we present new field and structural data from the MMR, the Monti Martani Fault System (MMFS), and the associated Medio Tiberino and Terni basins. We performed structural analyses of exposed fault surfaces deforming both the Mesozoic units and the Plio-Quaternary deposits. Aiming at constraining the local post-orogenic stress field at the time of faulting, we carried out paleostress analysis on extensional faults. Our field-based structural geology approach allows us to discuss the role of pre-orogenic structural inheritance on the Plio-Quaternary structuring of the area and to explore the possible relationships between post-orogenic extensional faulting and the Plio-Quaternary continental units to draw inferences on the current state of fault activity and its potential for generating fault displacement hazard.
2.1 The northern Apennines
The northern Apennines of Italy (Fig. 1) are a seismically active belt that results from a complex and polyphase evolution that started in the Mesozoic. It recorded a Jurassic rifting phase related to the opening of the Alpine Tethys, a later Late Cretaceous to Neogene orogenic phase related to Africa–Eurasia convergence, and a final post-orogenic extensional phase that has affected the internal part of the orogen since the Miocene (e.g., Boccaletti et al., 1971, 1985; Boccaletti and Guazzone, 1974; Principi and Treves, 1984; Vai and Martini, 2001; Molli, 2008; Conti et al., 2020, and references therein). Recent studies have also documented several episodes of synsedimentary extensional tectonic activity between the end of the Tethyan rifting and the beginning of the orogenic phase in the northern and central Apennines (e.g., Centamore et al., 2007; Cipriani and Bottini, 2019a, b; Capotorti and Muraro, 2021, 2024; Sabbatino et al., 2021).
The Jurassic rifting started in the Sinemurian, resulting in the dismembering of an extensive carbonate platform and the progressive separation of Europe from the Adriatic plate (e.g., Bernoulli et al., 1979; Ziegler, 1988). On the Adria margin, rifting led to the development of an articulated paleogeography with intrabasinal horsts and grabens leading to the deposition of pelagic successions in the grabens and of condensed sequences on pelagic carbonate platforms (PCPs) on horsts (e.g., Santantonio, 1993; Santantonio and Carminati, 2011; Cipriani et al., 2019, 2020; Santantonio et al., 2020). In the northern and central Apennines, particularly at their transition, the dominant strike of the Jurassic normal faults is ∼ N–S and ∼ E–W (e.g., Coltorti and Bosellini, 1980; Calamita et al., 1991; Cipriani et al., 2020). This inherited structural template was crucial for the localization of deformation during the subsequent orogenic phase through the positive inversion of the extensional pre-orogenic faults bounding the Jurassic horsts (e.g., Butler et al., 2004; Pizzi and Galadini, 2009; Scisciani, 2009; Pace and Calamita, 2014; Scisciani et al., 2014; Tavani et al., 2023; Curzi et al., 2024).
Starting from the Late Cretaceous, Africa–Eurasia convergence led to the progressive closing of the Alpine Tethys, which culminated in the Eocene–Oligocene continental collision leading to the structuring of the northern Apennine fold and thrust belt (e.g., Doglioni et al., 1999; Rosenbaum et al., 2004; Mantovani et al., 2009, and references therein). This contractional phase caused the progressive stacking of nappes of different paleogeographic domains from the former Adriatic margin of the Piemont-Liguria Ocean (e.g., Elter, 1975; Vai and Martini, 2001; Vezzani et al., 2010; Cosentino et al., 2010; Conti et al., 2020). The eastward migration of NE-verging thrusting and folding (that is orthogonal to the orientation of the present-day belt axis) controlled the structural development of the belt toward the foreland. This resulted in overthrusting of oceanic and transitional units, originally located farther west, on top of the external units (eastern side) belonging to the Adria microplate (Tuscan domain, Umbria–Marche domain).
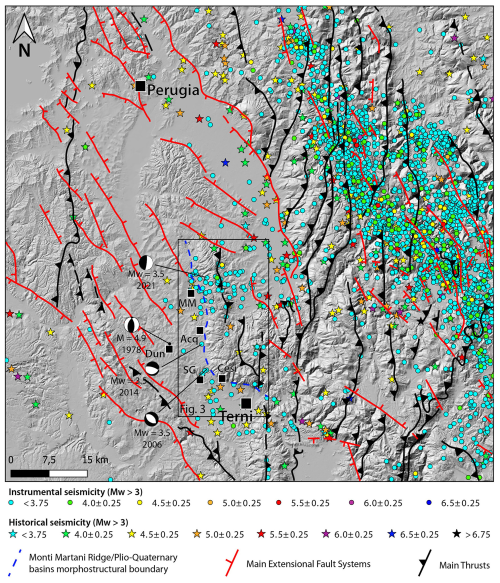
Figure 2Digital terrain model of the Monti Martani Ridge and surrounding region with locations of the epicenters of the instrumental (dots) and historical (stars) earthquakes with Mw > 3. Instrumental seismicity is from the INGV-ISIDe catalogue (see ISIDe Working Group, 2007). Historical seismicity is from the INGV-ASMI-CPTI catalogue (see Rovida et al., 2020, 2022). Earthquake focal mechanisms are from the INGV-TDMT catalogue (see Scognamiglio et al., 2006), with the exception of the 1979 M = 4.9 Dunarobba earthquake, which is from Gasperini et al. (1985). Main extensional fault systems and thrusts are from Calamita and Pierantoni (1995).
Starting in Miocene times, the internal part of the Apennines underwent post-orogenic extension, resulting in the opening of the Tyrrhenian Basin and of a series of intermontane marine to continental basins on the Tyrrhenian side of the belt (e.g., Malinverno and Ryan, 1986; Keller et al., 1994; Cavinato and De Celles, 1999; Faccenna et al., 1997, 2001; Rosenbaum and Lister, 2004; Patacca et al., 2008; Carminati et al., 2012). The extensional front progressively migrated E- and NE-ward through the activation of NW–SE-striking normal faults. This tectonic regime still governs most of the current seismicity (producing up to Mw = 7 events; ISIDe Working Group, 2007; Fig. 2) of the hinterland and axial regions of the Apennines (e.g., Cello et al., 1997; Mariucci et al., 1999; Boncio et al., 2000).
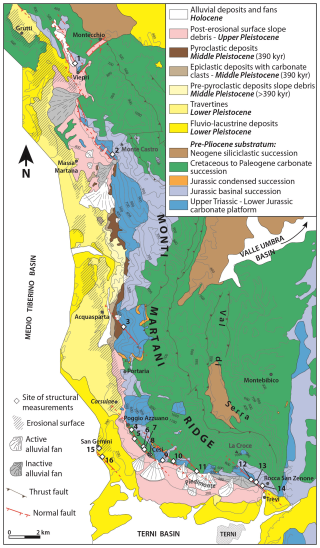
Figure 3Schematic geological map of the Martani Fault System (redrawn and modified after Bonini et al., 2003) with the location of our structural stations. Traces of normal faults have been schematically redrawn according to original field observations presented in this study. However, the full structural complexity of the MMR (i.e., within the ridge) is not reported here. The reader can refer to the geological maps by Regione Umbria – Servizio Geologico (2013).
2.2 The Monti Martani Ridge
The MMR is an ∼ N–S-trending, ∼ 40 km long, L-shaped mountain ridge located in the southern part of the northern Apennines. It separates the Medio Tiberino Basin to the W from the Umbria Valley Basin to the east (Figs. 1b, 2 and 3). To the south it is bounded by the Terni Basin. The ridge is composed of a Meso-Cenozoic carbonate platform transitioning upward to the pelagic deposits of the Umbria–Marche succession (sensu Centamore et al., 1986; Fig. 4) and a Miocene foreland basin succession (Accordi, 1966; Barchi, 1991; Brozzetti and Lavecchia, 1995; Fig. 3). The overall structural architecture of the ridge consists of an E-verging anticline (in the west), exposing the Meso-Cenozoic carbonate to marly succession and thrusting towards the ∼ E on an ∼ N–S-trending syncline cored by Oligo-Miocene turbiditic units (Alfonsini et al., 1991; Calamita and Pierantoni, 1994, 1995; Alfonsini, 1995; Brozzetti and Lavecchia, 1995). On top of the Hettangian–Sinemurian platform carbonate (Calcare Massiccio Fm.), the Jurassic stratigraphic succession reveals an articulated paleogeography. In fact, the typical pelagic to hemipelagic Jurassic units of the complete Umbria–Marche succession accumulated in the hanging wall basins and are locally replaced by the condensed succession of the Bugarone group, which deposited on top of Jurassic horsts (Mariotti et al., 1979; Farinacci et al., 1981; Barchi et al., 1991; Brozzetti and Lavecchia, 1995; Bruni et al., 1995; Galluzzo and Santantonio, 2002; Fig. 4). Cipriani et al. (2020) therefore proposed that the Monti Martani area was a Jurassic pelagic carbonate platform, suggesting that the structural trends of the modern MMR (i.e., ∼ N–S and ∼ WNW–ESE) are directly inherited from the Jurassic rifting phase. Indeed, Jurassic faults have been documented to trend N–S, N20°, E–W, and N110° (Bruni et al., 1995). Moreover, a significant influence of pre-Pliocene ∼ N–S- and ∼ W–E-trending structural features has been reported from the easternmost sector of the MMR (Montebibico area) and has been attributed to the inherited structural template of the Jurassic extension (Coltorti et al., 1995).
The present-day regional stress field of the internal part of the northern and central Apennines relates to an extensional tectonic regime with a minimum horizontal stress axis (Shmin) oriented NE–SW (Mariucci and Montone, 2024, and references therein). However, local deviations are reported from the Monti Martani area, where focal mechanisms of extensional earthquakes with an Shmin oriented N–S or E–W have been reported (Pondrelli et al., 2006).
2.3 The Monti Martani Fault System
The MMR is bounded to the west and to the south by the MMFS (also referred to in the literature as Faglia Martana by Brozzetti and Stoppa, 1995; Faglia dei Monti Martani by Brozzetti and Lavecchia, 1995; and Martana Fault by Bonini et al., 2003), an extensional fault system separating the Meso-Cenozoic carbonate succession from the Plio-Quaternary Medio Tiberino (to the west) and Terni (to the south) basins. In the literature, this fault system is described as a single L-shaped structure, with an ENE-facing concavity, including an ∼ 30 km long N–S- to NNW–SSE-striking segment extending from Grutti to San Gemini and an ∼ 10 km long WNW–ESE-striking segment extending from Cesi to Trevi (Brozzetti and Lavecchia, 1995; Bonini et al., 2003). Although this fault system is commonly represented in the literature as a continuous fault, with the two segments merging at the southwestern tip of the MMR, its surface expression is much more complex and ambiguous and does not necessarily match the morphostructure of the western and southern flanks of the MMR (see below). The cumulative vertical displacement across the MMFS has been estimated to be ∼ 2000 m on a seismic cross-section across the northern tip of the MMR, even though the thickness of the associated Medio Tiberino Basin is only ∼ 500 m (Barchi et al., 1991). Bonini (1998) proposed that the MMFS developed as an extensional reactivation of the ramp of an earlier Monti Martani thrust.
The Medio Tiberino Basin is an N–S- to NNW–SSE-trending graben to the west of the MMR and is filled by an ∼ 500 m thick Upper Pliocene–Quaternary continental succession (Conti and Girotti, 1977; Ambrosetti et al., 1978, 1987a; Barchi et al., 1991; Basilici, 1997). The lower part of the succession consists of Upper Pliocene–Lower Pleistocene fluvio-lacustrine clay, sand, and gravel, overlain by ∼ 50 m thick Lower Pleistocene bedded travertine (Barchi et al., 1991). These deposits are locally overlain by slope debris and volcanic/volcanoclastic deposits (Brozzetti and Stoppa, 1995; Bonini et al., 2003) dated to the Middle Pleistocene by the 39Ar/40Ar method (0.39 ± 0.01 Ma; Laurenzi et al., 1994). This part of the succession is modeled by a planation surface which was eroded during the Middle Pleistocene (Bonini et al., 2003) and has a smooth N–S topographic gradient. Reddish Upper Pleistocene slope debris postdates the erosional surface and crops out extensively along the western and southern slopes of the MMR (Bonini et al., 2003). The Terni Basin is an E–W-trending graben exposed to the south of the MMR and is considered the southeastern branch of the Medio Tiberino Basin, with which it shares a common stratigraphic evolution (Basilici, 1993; Cattuto et al., 2002). To the north, the Terni Basin terminates against the ∼ ESE-trending slopes of the southern termination of the MMR.
The Parametric Catalogue of Italian Earthquakes – CPTI (Rovida et al., 2020, 2022) contains few historical earthquake epicenters with Mw > 4 along the MMFS, with the strongest being the 5.1 Mw 1751 CE Terni earthquake and no historical earthquake reported in the N–S-striking segment of the fault system (Fig. 2). Instrumental seismicity recorded since 1985 (ISIDe Working Group, 2007) highlights moderate seismic activity (Mw ≤ 3.5) along the MMFS, with two main clusters located in the Massa Martana and Cesi/San Gemini sectors (Fig. 2). Only three focal mechanisms have been resolved along the MMFS for 3.5 Mw earthquakes (Scognamiglio et al., 2006), and they show inconsistent fault plane solutions: while the 2006 San Gemini earthquake yields an extensional mechanism with a horizontal NE–SW extension direction compatible with the current regional stress field, the 2014 Acquasparta earthquake yields an ∼ N–S extension with a slightly oblique dextral component, and the 2021 Massa Martana earthquake is associated with a compressional mechanism with N–S-striking planes and a W-plunging P axis (Fig. 2). Although the latter event seems totally incongruous with the overall regional stress field, it is quite consistent with the N–S-striking planes with a sub-horizontal E–W-trending P axis of the focal mechanism reconstructed for the 1978 Dunarobba earthquake on the western margin of the Medio Tiberino Basin (Gasparini et al., 1985).
Despite the complexity of this seismic framework, only the ∼ ESE-striking segment bounding the northern margin of the Terni Basin has been proposed as a potential seismogenic fault (Lavecchia et al., 2002). However, both segments of the MMFS are regarded as active and capable faults in the ITaly HAzard from CApable faults (ITHACA) catalogue (ITHACA Working Group, 2019). Accordingly, Bonini et al. (2003) suggested that the MMFS cuts through Upper Pleistocene slope debris and displaces the archeological ruins of the Roman town of Carsulae. However, this interpretation was challenged by later archeological studies (Aringoli et al., 2009; Bottari and Sepe, 2013).
In this work, we used a field-based approach to characterize fault geometry and kinematics and to make inferences on the relationships between faulting and deposition of the Plio-Quaternary basin infill. In particular, we focused our attention on mapping the faults exposed along the morphological boundary between the MMR and the Medio Tiberino and Terni basins (i.e., along the trace of the supposed L-shaped Martana Fault System; Fig. 3). We defined their orientation, geometry, kinematics, and, when possible, cross-cutting relationships between fault populations. Most of the measurements were made in the Mesozoic carbonate succession of the MMR (i.e., in the footwall of the supposed L-shaped MMFS), while only a few structural stations were studied in the Plio-Quaternary basin fill due to a substantial lack of exposure (Fig. 3; Table S1 in the Supplement).
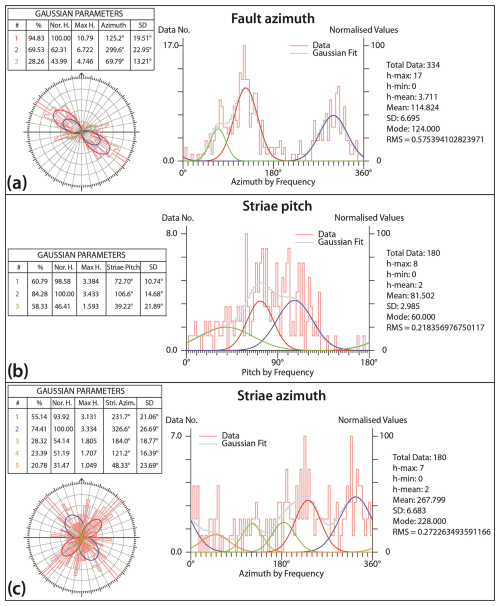
Figure 5Cumulative fault data collected in this study. Polymodal Gaussian distribution statistics of the cumulative fault azimuth (a) and the slickenside striations pitch (b) and azimuth (c). Statistical analysis was performed using the Daisy v.4.1 software (Salvini et al., 1999). The Gaussian parameters are as follows: number of Gaussian peak (#), percentage of occurrence (%), normalized height (Nor.H.), maximum height (Max.H.), and standard deviation (SD).
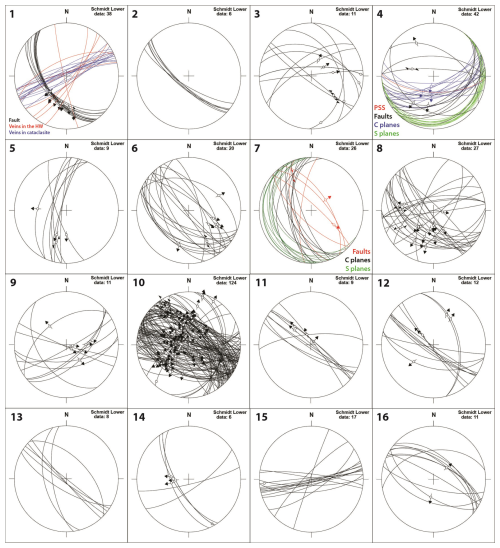
Figure 6Equal-area stereoplots (Schmidt net, lower hemisphere) showing the attitude of the main structural features measured in the field and drawn with the Win-Tensor program (Delvaux, 1993). Numbers refer to the sites of structural measurements shown in Fig. 3.
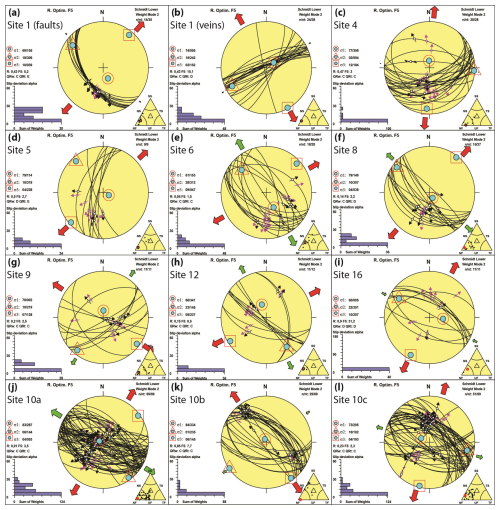
Figure 7Paleostress tensors calculated from the inversion of fault slip data using the Win-Tensor software (Delvaux, 1993). Stereograms are Schmidt lower-hemisphere projections. In the stereograms, black arrows represent the measured hanging-wall slip vector on a fault plane; purple arrows represent the theoretical optimal slip vector on a fault plane with respect to the computed paleostress tensor. Site numbers refer to numbers of the structural stations in Fig. 3. In the Frohlich triangles, NF is pure normal faulting, TF is pure reverse faulting, SS is pure strike-slip faulting, NS is transtension, TS is transpression, and UF is radial extension.
Statistical analysis of fault and striae populations in the cumulative fault dataset was carried out with the Daisy v.4.1 software (Salvini et al., 1999; Fig. 5). Structural data were plotted for each structural station (Fig. 6) on a Schmidt net, and lower-hemisphere stereographic projections were drawn with the Win-Tensor program (Delvaux, 1993). When sufficiently abundant and statistically meaningful, these data were used to perform paleostress analysis to reconstruct the orientation of the paleostress tensor for each structural station (Fig. 7). Paleostress analysis was performed with the Win-Tensor program (Delvaux, 1993), which uses a refined version of the Right Dihedron method (Angelier and Mechler, 1977) and an iterative Rotational Optimization procedure (Delvaux and Sperner, 2003). Data separation for tensorial analysis followed the workflow described in Mattila and Viola (2014) and was first performed based on field observations (i.e., cross-cutting relationships between faults or separation of different kinds of structures, such as faults, veins, and cleavage planes) and on a qualitative kinematic analysis of the stereonets (i.e., definition of striae trend populations). We then adopted an iterative approach by using the Right Dihedron method and the Rotational Optimization procedure in sequence to compute a paleostress tensor while progressively removing those data that turned out to be kinematically incompatible with the calculated stress model. In particular, we removed all data with a slip deviation angle α > 30°, which is defined as the acute angle between the measured slip vector on a fault plane and the theoretical optimal slip vector on the same fault plane with respect to the computed paleostress tensor. This parameter determines the robustness of the optimization procedure, since faults with α≤ 30° are regarded as compatible with the calculated reduced paleostress tensor.
4.1 Cumulative fault data analysis
Merging of all fault datasets from the different structural stations allowed us to perform a cumulative statistical analysis on fault data at the scale of the study area (Fig. 5). Distribution of fault azimuth reveals that there are two major trends in fault strike (Fig. 5a). The dominant trend is represented by a vast majority of NW–SE-striking faults (N125° and N300°), while a second subsidiary fault population is composed of fault planes striking ENE–WSW (N070°). Neither of these two trends correlates with the orientation of the morphological boundary between the MMR and the Medio Tiberino and Terni basins (i.e., ∼ N–S and ∼ N100°, respectively).
Three pitch populations have been found for the slickenside striations measured on fault surfaces (73, 107, and 39°; Fig. 5b). This distribution documents dominant dip-slip over subordinate strike-slip kinematics. Analysis of striae azimuth distribution on normal, oblique/normal, and strike-slip faults identified five different populations (N232°, N327°, N184°, N121°, and N048°; Fig. 5c). These populations define three main directions of movement along fault planes: NE–SW (populations 1 and 5 in Fig. 5c), NW–SE (populations 2 and 4 in Fig. 5c), and N–S (population 3 in Fig. 5c).
4.2 Fault data and paleostress analysis on single structural stations
In the following, we describe the key structural features observed at selected field stations along the trace of the supposed L-shaped MMFS, starting from its northernmost tip and progressing towards its southeastern end (Fig. 3).
The Viepri site (structural site no. 1 in Fig. 3) is characterized by an SW-dipping normal fault that separates the Jurassic micritic limestones of the Corniola Fm., in the footwall, from the Eocene marls of the Scaglia Variegata Fm., in the hanging wall (Fig. 8). The principal slip surface (PSS) of the fault is marked by a fine-grained cohesive cataclasite formed at the expense of the Jurassic carbonate (Fig. 8), while, above the PSS, a layer of cataclasite and proto-cataclasite a few meters thick was formed at the expense of the Eocene marly lithotypes. These latter have a coarser grain size and are less consolidated than those of the PSS and contain an extensional top-to-the-SW S-C fabric (sensu Berthé et al., 1979). Slickenside striations on the PSS point to a normal to normal/oblique sense of shear with a sinistral component (stereonet no. 1 in Fig. 6). Cataclasites in the hanging wall of the PSS and, locally, the PSS itself, are cut by centimeter- to decimeter-thick NE–SW-trending sub-vertical calcite veins (Fig. 8b). Sub-vertical calcite veining also occurs in marls of the Scaglia Variegata Fm. in the hanging wall of the fault, though with a more diffuse and pervasive spatial distribution (Fig. 8c). The paleostress tensor reconstructed with data from the PSS shows a sub-horizontal NE–SW-trending σ3 axis (Fig. 7a), while the opening of tensile veins in the hanging wall of the faults is compatible with an NW–SE-trending σ3 axis (Fig. 7b).
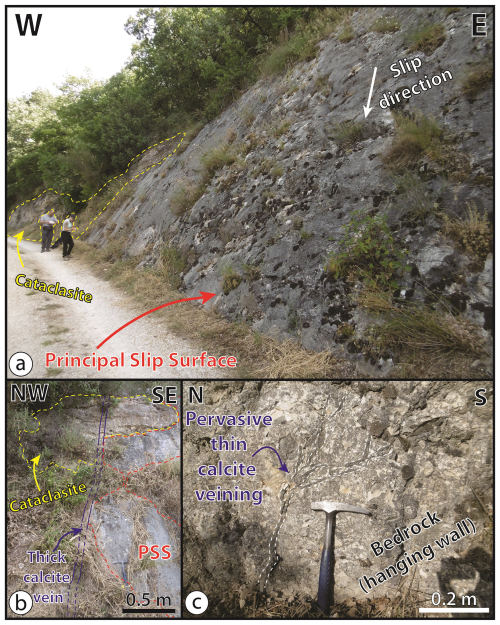
East of Massa Martana, on the western slope of the Monte Castro (structural site no. 2 in Fig. 3), mesoscale NW–SE-trending normal faults cut through the Lower Jurassic limestone of the Calcare Massiccio Fm. (stereonet no. 2 in Fig. 6). Due to the lack of striae on the fault surfaces, the dataset is too small for a reliable paleostress analysis here. However, at the first order, the strike of these few faults is generally compatible with an NE–SW-trending direction of extension.
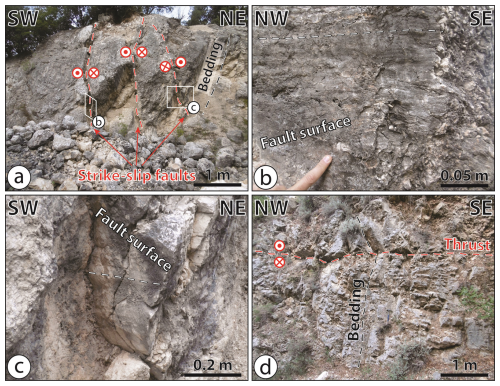
Figure 9Examples of synorogenic structures in the Calcare Massiccio Fm. in the Acquasparta quarry (site no. 3 in Fig. 3; stereonet no. 3 in Fig. 6). (a) Sub-vertical, NE–SW-, and NW–SE-striking strike-slip faults (marked by dashed red lines), with details of two fault surfaces (in panels b and c) with strike-slip slickenlines marked by dashed white lines. (d) NW–SE-trending thrust fault marked by the dashed red line. Note the diffuse brecciation and fracturing at the outcrop scale.
In the abandoned quarry to the east of Acquasparta (structural site no. 3 in Fig. 3), the Calcare Massiccio Fm. appears to be highly fractured and cut by an intricate tangle of faults, including normal/oblique and sub-vertical sinistral fault planes (stereonet no. 3 in Figs. 6 and 9). Intense fracturing at the outcrop scale appears to be correlated with diffuse strike-slip and reverse faulting likely related to the orogenic contractional phase. Consequently, it is hard here to distinguish if the oblique/extensional planes are neoformed or reactivated inherited slip surfaces. At this site, our difficulty in identifying proper fault clusters related to specific tectonic events precluded a reliable paleostress analysis.
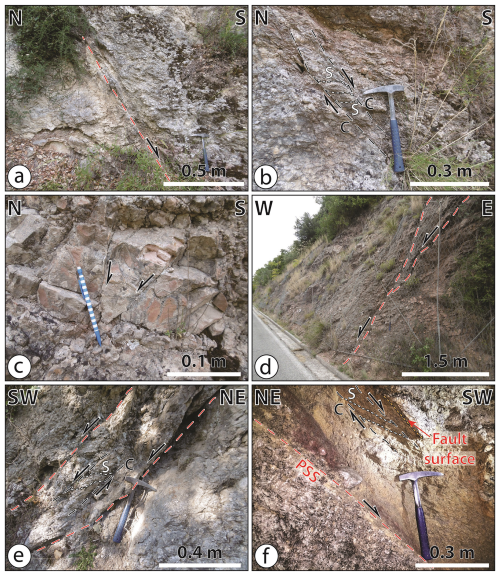
Figure 10(a) S-dipping PSS of a normal fault cutting through the Scaglia Rossa Fm. in the Poggio Azzuano area (site no. 4 in Fig. 3; stereonet no. 4 in Fig. 6), with detail of the extensional top-to-the-S S-C fabric developed within the cataclasite (b) and of a second-order conjugate normal faults pair (c) in the hanging wall of the PSS. (d) Example of a W-dipping normal fault cutting through the Scaglia Rossa Fm. E of Poggio Azzuano (site no. 5 in Fig. 3; stereonet no. 5 in Fig. 6). (e) Extensional top-to-the-SW S-C fabric within foliated cataclasite formed at the expense of the Corniola Fm. cropping out W of Cesi (site no. 7 in Fig. 3; stereonet no. 7 in Fig. 6). (f) Extensional SW-dipping PSS putting the Calcare Massiccio Fm. (in the footwall) in contact with the Rosso Ammonitico Fm. (in the hanging wall) in the Grotta Eolia site in Cesi (site no. 8 in Fig. 3; stereonet no. 8 in Fig. 6); note the top-to-the-SW extensional S-C fabric developed within the fine-grained cataclasite of the fault core and a second-order SW-dipping fault surface with dip-slip slickenlines (marked by the thin dashed orange line) in the hanging wall of the PSS.
In the Poggio Azzuano area (structural site no. 4 in Fig. 3), at the southernmost tip of the N–S-trending segment of the boundary between the MMR and the Medio Tiberino and Terni basins, an S-dipping PSS is found along with extensional top-to-the-S S-C structures within the marly limestone of the Cretaceous Scaglia Rossa Fm. (stereonet no. 4 in Figs. 6 and 10a–c). The paleostress tensor calculated at this site shows an NNE–SSW direction of extension (Fig. 7c). About 500 m to the SSE of this site, along the road to Cesi (structural site no. 5 in Fig. 2), the computed paleostress tensor is substantially different. The Scaglia Rossa Fm. is cut there by N–S normal to normal/oblique faults with a sinistral component (stereonet no. 5 in Figs. 6 and 10d). Paleostress determination from this fault system yielded an extensional regime with an NE–SW-trending sub-horizontal σ3 axis (Fig. 7d), compatible with that reconstructed from the Viepri site.
In the western part of the Cesi village (structural site no. 6 in Fig. 3), steeply dipping extensional fault planes cut through cohesive cataclasites formed at the expense of the fine-grained limestone of the Corniola Fm. All these faults strike NW–SE and dip either to the NE with oblique normal/dextral striae or to the SW with oblique normal/sinistral striae (stereonet no. 6 in Fig. 6). Tensorial analysis performed on this dataset yielded an NE–SW-trending sub-horizontal σ3 axis, with a steeply dipping σ1 axis and a gently dipping σ2 axis (Fig. 7e). In this same area (structural site no. 7 in Fig. 3), foliated cataclasites in the Corniola Fm. display a penetrative S-C fabric (stereonet no. 7 in Figs. 6 and 10e). This dataset did not allow us to perform a proper tensorial analysis. However, the attitude of the S-C fabric is consistent with an extensional tectonic regime with an NE–SW-trending direction of extension.
At the karstic cave of the Grotta Eolia site in the Cesi village (structural site no. 8 in Fig. 3), a complex network of faults belonging to an extensional fault system juxtaposes the Calcare Massiccio Fm. in the footwall against cataclastic red marly limestone in the hanging wall (stereonet no. 8 in Figs. 6 and 10f). Rocks in the hanging wall can be tentatively attributed to the Rosso Ammonitico Fm. Variably oriented high- and low-angle faults inherited from the previous orogenic phase are also present in the cave. NW–SE-striking normal to oblique/normal faults, with striae pitch values between ∼ 70° and ∼ 100° (Fig. 10f), are compatible with an extensional paleostress tensor with an NE–SW-trending sub-horizontal σ3 axis (Fig. 7f), as are the other two sites in the western part of the Cesi village described above (structural sites no. 6 and 7 in Fig. 6). By contrast, a substantially different paleostress tensor has been obtained for NE–SW-striking extensional faults exposed ∼ 1 km E of Cesi (structural site no. 9 in Fig. 3) and cutting through the micritic limestone of the Maiolica Fm. (stereonet no. 9 in Fig. 6). There, paleostress analysis resulted in an Andersonian extensional system with an NW–SE-trending direction of extension (Fig. 7g). This paleostress tensor is consistent with that responsible for the formation of the tensile veins at the Viepri site (site no. 1 in Figs. 6 and 7b).
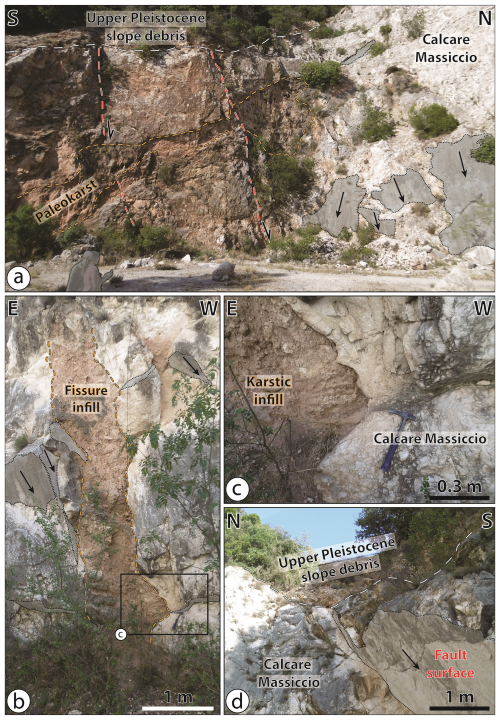
Figure 11Outcrop pictures from the Cesi quarry (site 10 in Fig. 3). Structural measurements from this site are reported in stereonet no. 10 in Fig. 6. Red arrows indicate extensional fault planes in the Calcare Massiccio Fm. (a) Panoramic view of the western wall of the quarry, with a south-dipping paleo-karstic network with speleothems developed in the faulted limestone of the Calcare Massiccio Fm.; note that Upper Pleistocene slope debris sutures normal fault planes. (b) Example of a sub-vertical, non-faulted Quaternary fissure infill within the Calcare Massiccio Fm. (c) Detail of the contact between a fissure infill and the Calcare Massiccio Fm. (see Fig. 5b for location); note that the faults and fractures affecting the limestone do not propagate within the karstic infill of the fissure. (d) Example of Upper Pleistocene slope debris suturing a normal fault cutting through the Calcare Massiccio Fm. Shaded gray polygons show fault surfaces, with black arrows showing the slip direction of the hanging wall block.
About 1 km to the east of Cesi, the limestone of the Calcare Massiccio Fm. exposed in an abandoned quarry (structural site no. 10 in Fig. 3) appears intensely fractured and cut by a dense network of high-angle normal, oblique, and strike-slip faults (Fig. 11 and stereonet no. 10 in Fig. 6). Some NE-dipping fault planes bear two sets of slickenlines, one related to normal to oblique slip (pitch = 60–70°) and one related to strike-slip (pitch ∼ 5–25°), with the latter post-dating the first (Fig. 12). We performed tensorial analysis on a dataset of more than 100 fault planes, most of which strike NW–SE. After data treatment, we distinguished three data subsets: (i) one including normal/oblique fault planes with NE–SW-trending slickenlines, (ii) one characterized by sinistral strike-slip faults with NW–SE-trending slickenlines, and (iii) one with normal/oblique (with a sinistral component) fault planes with NNE–SSW-trending slickenlines (Fig. 7j–l, respectively). Tensorial analysis performed on these three data subsets yielded three different Andersonian extensional paleostress tensors, with a sub-vertical σ1 axis and a sub-horizontal σ3 axis trending NE–SW, NW–SE, and NNE–SSW, respectively (Fig. 7j–l). All these results have a satisfying slip deviation angle α < 30°. It is worth noting that these three paleostress tensors reconstructed from this large dataset in a single location are consistent with those calculated in other sites along the MMFS, where datasets are smaller and tensorial analysis only gave one paleostress tensorial solution for each site.
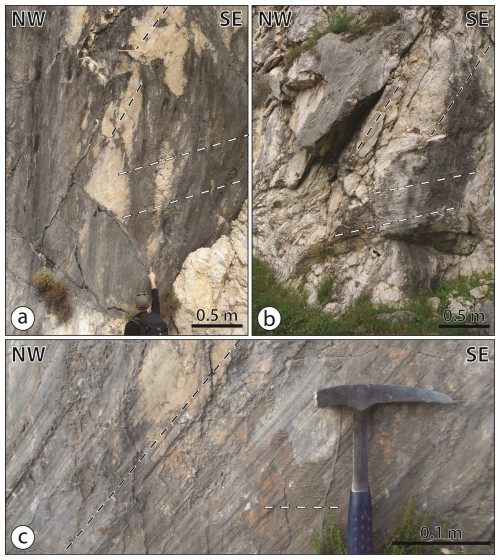
Sub-vertical NW–SE-striking normal/oblique fault planes in the Calcare Massiccio Fm. have also been measured close to the Madonna dell'Olivo Sanctuary in the Piedimonte area (structural site no. 11 in Fig. 3; stereonet no. 11 in Fig. 6). Unfortunately, the dispersion of planes is too small in this dataset to perform a reliable tensorial analysis. At this same location, Brozzetti and Lavecchia (1995) and Bonini et al. (2003) reported mainly E–W- to WNW–ESE-striking normal/oblique (dextral) faults, compatible with an extensional paleostress tensor with a sub-horizontal NE–SW σ3 axis (Brozzetti and Lavecchia, 1995).
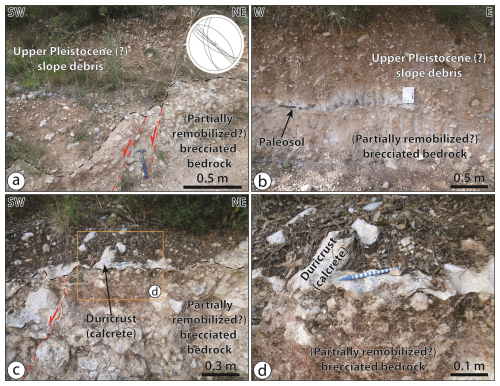
Figure 13Outcrops in the Fontana della Madonna area (site no. 13 in Fig. 3). (a) Example of extensional faults cutting through the Mesozoic carbonate substratum and being sutured by Upper Pleistocene (?) slope debris, with stereographic projection (Schmidt net, lower hemisphere) of fault data from this site. (b) Dark-brown paleosol at the contact between the brecciated Mesozoic bedrock and the overlying Upper Pleistocene slope debris. (c, d) Carbonate duricrust (calcrete) locally hardening the paleosol between the brecciated Mesozoic bedrock and the overlying Upper Pleistocene slope debris and suturing normal faults cutting through the carbonate substratum.
At structural site no. 12 (Fig. 3), NW–SE-striking extensional fault planes cut through the limestone of Calcare Massiccio Fm. (stereonet no. 12 in Fig. 6). The paleostress tensor reconstructed at this site has an NE–SW-trending sub-horizontal σ3 axis (Fig. 7h), even though the σ1 axis is not exactly sub-vertical (dip angle 66°). An analogue set of faults has been measured at structural site no. 13 (Fig. 3; stereonet no. 13 in Fig. 6). These extensional faults cut through the brecciated Corniola and are sutured by Upper Pleistocene slope debris (Fig. 13). The brecciated Corniola Fm. consists of a monomictic, angular, and heterometric breccia not exceeding 2–3 m in thickness. The clasts are derived from the underlying bedrock and exhibit a chaotic fabric, suggesting that the breccia formed locally (in situ), although a limited remobilization of clasts cannot be entirely ruled out. Unfortunately, no slickenlines were observed at this site, thus preventing tensorial analysis.

A similar situation has been observed in the easternmost structural site at the eastern tip of the MMFS (Rocca San Zenone area, structural site no. 14 in Fig. 3), where extensional, oblique, and strike-slip faults cut through the brecciated Corniola Fm. and are sutured by Upper Pleistocene slope debris (stereonet no. 14 in Fig. 6). On a single NW–SE-striking and SW-dipping fault plane, three different generations of striae have been observed, pointing to a polyphase reactivation of this fault plane with a normal, oblique (normal/dextral), and strike-slip movement (Fig. 14). However, the dispersion of planes in this dataset is too small to generate a reliable tensorial analysis.
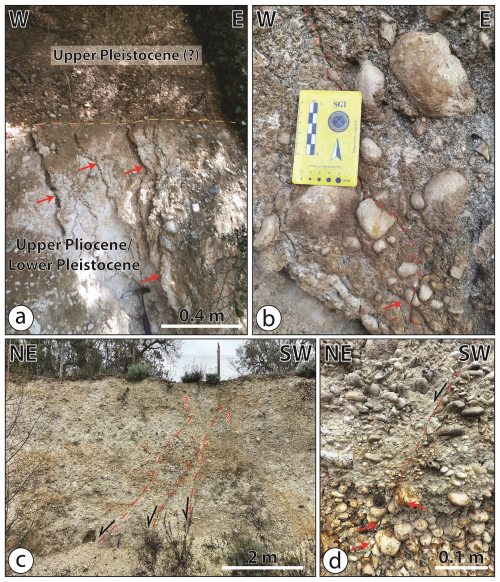
Figure 15Examples of brittle structures within Upper Pliocene/Lower Pleistocene yellowish conglomerates in the San Gemini area. (a) Sub-vertical fractures (indicated by red arrows) within Upper Pliocene/Lower Pleistocene conglomerates sutured by Upper Pleistocene (?) reddish alluvial conglomerates in San Gemini (site 15 in Fig. 3; stereonet no. 15 in Fig. 6). (b) Detail of an irregular sub-vertical fracture within Upper Pliocene/Lower Pleistocene yellowish conglomerates at the same site as panel (a); the red arrow points to a broken pebble straddling the fracture plane. (c) NE-dipping normal faults within Upper Pliocene/Lower Pleistocene conglomerates exposed in an abandoned quarry south of San Gemini (site 16 in Fig. 3; stereonet no. 16 in Fig. 6); note that upward fault throw appears progressively less evident. (d) Detail of an NE-dipping normal fault in the same site as panel (c); note the broken pebbles marked by red arrows in the downward continuation of the fault plane (marked by a dashed red line).
As to extensional deformation in the Plio-Quaternary fill of the Medio Tiberino and Terni basins, brittle structures were only observed in the San Gemini area cutting through Upper Pliocene/Lower Pleistocene yellow sandy conglomerates, likely belonging to the Fosso Bianco Fm. sensu Basilici (1992, 1997) (Fig. 15). These structures consist of sub-vertical fractures within the San Gemini area (structural site no. 15 in Fig. 3; stereonet no. 15 in Fig. 6) and normal faults exposed in an abandoned quarry south of San Gemini (structural site no. 16 in Fig. 3; stereonet no. 16 in Fig. 6). Tensorial analysis performed on this latter fault set provided an extensional paleostress tensor with a sub-horizontal NE–SW-trending σ3 axis (Fig. 7i).
4.3 Relationships between extensional faults and the Plio-Quaternary sediments
In this study, for the Plio-Quaternary age of the continental sedimentary units of the Medio Tiberino and Terni basins, we have adopted the stratigraphic schemes of Basilici (1997), Bonini et al. (2003), and the 1:10.000-scale geological map of the Regione Umbria – Servizio Geologico (2013). All these studies agree on the age of the deposits described in the San Gemini area and along the western and southern margins of the MMR. The majority of the studied fault surfaces along the MMFS cut through the Meso-Cenozoic carbonate sequences of the MMR. Very few faults have been documented in the Plio-Quaternary infill of the Medio Tiberino and Terni basins, and in no cases have faults been observed cutting through deposits younger than the Upper Pliocene/Lower Pleistocene. In the rare cases where the relationships between continental deposits and fault planes can be clearly observed, the former appears to suture the faults. This is clearly visible at two sites located along the eastern part of the ESE-trending segment of the slopes of the MMR in the Fontana della Madonna and Rocca San Zenone areas (sites 13 and 14 in Fig. 3). There, Upper Pleistocene slope debris deposits suture NW–SE-striking normal to oblique faults cutting limestones of the Meso-Cenozoic substratum (Fig. 13a). In particular, in the Fontana della Madonna area (site no. 13 in Fig. 3), a dark-brown paleosol developed at the transition between the brecciated Mesozoic carbonate bedrock and the overlying Upper Pleistocene (?) slope debris (Fig. 13b). This paleosol is locally capped by a carbonate duricrust (calcrete) that sutures SW-dipping normal faults cutting through the brecciated Mesozoic carbonate substratum (Fig. 13c and d). Moreover, in an abandoned quarry to the east of Cesi, a paleo-karstic network is exposed (Fig. 11a). The orientation of the paleo-karstic system seems structurally controlled by NW–SE-trending normal to oblique faults. However, we observed neither deformation on the exposed speleothems or in the karst-filling sediments (Fig. 11b and c) nor displacement within the overlying poorly consolidated Upper Pleistocene reddish slope debris (Fig. 11d). No deformation has been observed to affect the speleothems of the Grotta Eolia, either (structural site no. 8 in Fig. 3).
Although extensional fault systems cutting through the oldest deposits of the Plio-Quaternary sedimentary infill and compatible with an NE–SW direction of extension are reported in the literature (Brozzetti and Lavecchia, 1995; Brozzetti and Stoppa, 1995; Basilici, 1997), very little evidence of deformation has been found in the continental deposits of the Medio Tiberino and Terni basins, mainly due to the general lack of informative outcrops. Most of the sites reported in the literature are actually located in ancient or still-active quarries. The only clear field evidence of Quaternary deformation is in the southern part of San Gemini (structural sites 15 and 16 in Fig. 3). There, E–W-trending sub-vertical joints cut through well-consolidated Upper Pliocene/Lower Pleistocene yellowish conglomerates (Fig. 15a and b; stereonet no. 15 in Fig. 6). These discontinuities are in turn sutured by poorly consolidated Upper Pleistocene (?) reddish alluvial conglomerates (Fig. 15a). Moreover, in an abandoned quarry right to the south of San Gemini, NW–SE-striking normal faults cut through the Upper Pliocene–Lower Pleistocene conglomerate (stereonet no. 16 in Figs. 6 and 15c and d). Some of these faults are characterized by a progressive upward reduction in the vertical throw, thus suggesting a synsedimentary fault activity (Fig. 15c).
5.1 Discrepancy between the morphostructural trends and the strike of single fault segments
After compiling the structural data along the trace of the MMFS, a striking observation is that there is no evident correlation between the morphostructural trend of the western and southern margins of the MMR and the strike of the measured mesoscale structures. In fact, in only very few cases does the strike of the measured extensional faults correlate with the ∼ N–S or WNW–ESE trends of the main inferred segments of the MMFS. The dominant measured fault strike is NW–SE (Fig. 5a). Moreover, ∼ E–W-striking faults exist along the ∼ N–S segment of the fault system (as in the case of the Poggio Azzuano area; structural site no. 4 in Fig. 3). Also, ∼ N–S-striking faults occur along the WNW–ESE segment (as in the case of the Cesi or Rocca San Zenone areas; structural sites no. 8, 12, and 13 in Fig. 3). This suggests, therefore, that the morphostructural trend of the western and southern margins of the MMR does not correspond to continuous and several-kilometer-long fault traces aligned along the ∼ N–S or WNW–ESE directions. Rather, the MMFS appears to be formed of several disconnected fault segments with different orientations, most of which are aligned with the main structural trend of the Plio-Quaternary extensional structures of the northern and central Apennines (i.e., NW–SE; e.g., Galadini and Galli 2000; Barchi, 2010).
Thus, our results show that the contact between the Upper Pleistocene–Holocene deposits of the Medio Tiberino and Terni basins and the Meso-Cenozoic carbonate and siliciclastic rocks of the MMR is an unconformable stratigraphic contact and not an extensional synsedimentary tectonic contact as proposed by earlier studies (e.g., Ambrosetti et al., 1987b; Bonini et al., 2003). In other words, we propose that the MMFS somehow steered the deposition of (at least part of) the Plio-Quaternary infill of the Medio Tiberino and Terni basins, although this does not imply that this tectonic control was exerted by one single major fault bounding the MMR.
In addition to the geometric misfit between the morphostructure at the large scale and the faults observed in the field as per the discussion above, the distribution of the epicenters of the major historical and instrumental earthquakes from the area also corroborates this apparent misfit, as they seem not to align along the morphostructural boundary between the MMR and the Medio Tiberino and Terni basins (ISIDe Working Group, 2007; Rovida et al., 2020, 2022). At least two clusters of epicenters can be identified east of Massa Martana, in the inner part of the MMR, and in the Terni area (Fig. 2). Moreover, the focal mechanisms of the 2006 San Gemini and 2014 Acquasparta earthquakes yield fault plane solutions that are misoriented to the main structural trend of the western boundary of the MMR (Scognamiglio et al., 2006; Fig. 2). In any case, the clustering of the instrumental epicenters in the study area is clearly not coherent with the supposed L-shaped geometry commonly attributed in the literature to the MMFS.
5.2 What does control the orientation of faults in the Monti Martani Ridge? The role of structural inheritance
If not governed by the active seismotectonics, the orientation of the main structural trends affecting the MMR and its L-shaped geometry may instead possibly be regarded as the long-lived expression of the inherited pre-orogenic structural grain. In particular, we refer to the regional-scale ∼ E–W- and ∼ N–S-trending tectonic lineaments that are thought to have controlled the Jurassic paleogeography in the Monti Martani, Sabina, and Monti Reatini areas after the dismembering of the Calcare Massiccio carbonate platform during the Early Jurassic rifting phase (e.g., Coltorti and Bosellini, 1980; Calamita et al., 1991; Cipriani et al., 2020). These structural trends match those of the Jurassic extensional faults described within the MMR (Bruni et al., 1995; Coltorti et al., 1995). Several studies have suggested that such structural inheritance may have influenced the nucleation and geometry of the main orogenic fronts during the Apennine nappe-stacking phase (e.g., Decandia and Tavarnelli, 1991; Tavarnelli, 1996; Butler et al., 2006; Scisciani, 2009, Scisciani et al., 2014; Tavarnelli et al., 2019; Curzi et al., 2024), including in the Monti Martani area (Calamita and Pierantoni, 1994, 1995; Bruni et al., 1995; Bonini, 1998). Remarkably, these studies have not reported any significant changes in the orientation of the pre-orogenic features that could be attributed to the synorogenic phase.
In light of these considerations, we suggest that, in this area, the pre-orogenic Jurassic tectonic grain might have exerted a fundamental structural influence not only on the Apenninic convergent phase but also on the Plio-Quaternary post-orogenic extensional phase, as suggested by recent studies in other portions of the Apennine belt (e.g., Tavani et al., 2021; Mercuri et al., 2024). This would agree with previous studies suggesting that the area of the Plio-Quaternary Terni Basin represented an early ∼ E–W-trending graben between the Monti Martani and the Sabina Plateau pelagic carbonate platforms already in the Jurassic (Cipriani et al., 2020). This depression may have been reactivated during the post-orogenic phase.
Numerical and analogue models of oblique rifting have shown that, in the presence of inherited structural features oriented at a substantial obliquity to the regional extension direction (i.e., ∼ 45°), the bulk large-scale morphostructural trend of the rift system is indeed governed by the orientation of the inherited structural grain rather than by the regional tectonic trends (e.g., McClay and White, 1995; Keep and McClay, 1997; Brune, 2014). These studies have also shown that the strike of the neoformed normal faults tends to be almost orthogonal to the regional σ3 direction (∼ 15° of deviation; see Brune, 2014). However, analogue models have also documented that, in the case of multiphase extension with different stretching direction, the impact of the inherited structures on the strike of the newly formed faults depends on the amount of extension during the first rifting phase (Wang et al., 2021). Numerical models have suggested that, in such cases, in the absence of variations in the orientation of the regional stress field during rifting, fault kinematics may evolve from a first stage dominated by normal faulting to a second stage where normal, oblique/normal, and strike-slip faulting coexist and where the strike of the faults may vary from almost orthogonal to the regional σ3 direction to broadly parallel to the direction of the inherited structural features (Brune, 2014, 2016). This implies that the orientation of locally derived paleostress tensors computed from limited fault slip data may deviate during rift evolution from the first-order regional stress field that determined the overall tectonic regime.
The setting described by these models is comparable to the context of the MMFS in the general tectonic framework of the transition between the northern and central Apennines. In fact, as discussed above, the MMR is characterized by significant structural inheritance due to the Jurassic-rifting-related ∼ E–W and ∼ N–S-striking faults. These structures have a moderate obliquity to the Quaternary and present-day regional σ3 direction related to post-orogenic extension in the internal part of the orogen that is NE–SW (e.g., Piccardi et al., 1997; Montone et al., 2012; Mariucci and Montone, 2024, and references therein). We thus suggest that the observed complex structural pattern of the MMFS might result from the interaction between inherited pre-orogenic structural features and post-orogenic regional stress tensor oriented obliquely to the pre- to synorogenic structural grain.
Mercuri et al. (2024) documented the reactivation of Jurassic normal faults belonging to an ∼ 10 km long fault system (Celano–Ovindoli–Pezza Fault System) in an internal portion of the central Apennines as extensional faults during post-orogenic extension. In that case, the strike of the inherited normal fault(s) (i.e., NNW–SSE) is only slightly oblique to the ideal strike of normal faults formed as optimally oriented in the framework of the post-orogenic extensional stress field; that is, NW–SE. This might have mechanically favored reactivation of pre-existing structures over the formation of neoformed optimally oriented faults. By contrast, in the case of the MMR, the strike of the inherited Jurassic faults (i.e., ∼ N–S and ∼ E–W; Bruni et al., 1995; Coltorti et al., 1995) exhibits moderate to high obliquity to the NW–SE direction, which would be the optimal direction for neoformed normal faults in the present-day extensional stress field. Thus, this might have favored neoformation of optimally oriented faults over reactivation of pre-existing misoriented faults (Fig. 16). This would agree with the observation that most of the faults documented in this study strike NW–SE (Fig. 5a) and that, accordingly, most of them have dip-slip slickenlines with oblique to strike-slip striae being clearly subordinate in our dataset (Fig. 5b). Also, the presence of a non-optimally oriented inherited structural grain seems to have favored the formation of a very segmented fault system composed of a patchwork of relatively short (a few kilometers long?), optimally oriented normal faults rather than the formation of long extensional faults, such as those known in other parts of the internal domain of the Apennines (Fig. 16). This interpretation might have relevant implications on seismic hazard assessments, since the length of seismogenic sources correlates linearly to the maximum potential magnitude of earthquakes (e.g., Wells and Coppersmith, 1994).
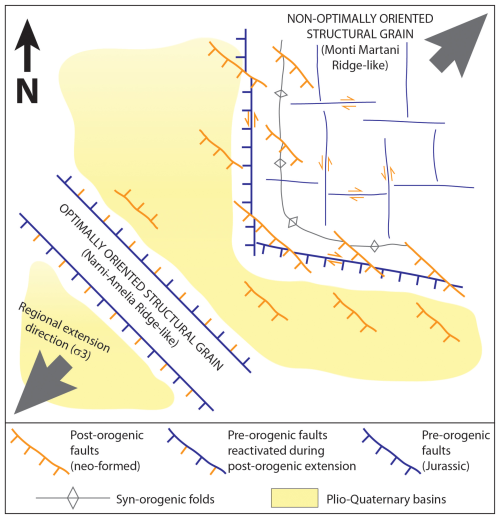
Figure 16Conceptual model for the development of post-orogenic extensional faults in the Monti Martani area. Optimally oriented structures are preferentially reactivated during post-orogenic extension and favor the formation of longer seismogenic normal faults. By contrast, a non-optimally oriented inherited structural grain with respect to the regional extensional stress field favors the activation of shorter, neoformed normal faults which are approximately perpendicular to the regional extension direction.
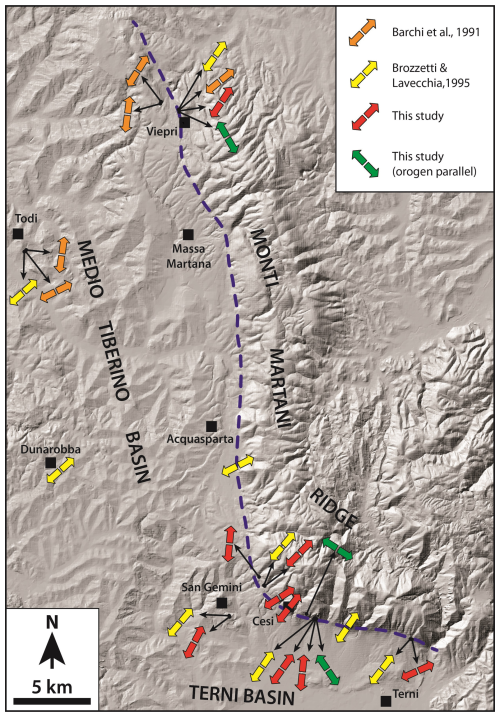
Figure 17Extension directions calculated after paleostress analyses of Plio-Quaternary faults of the Martani Fault System represented on a DTM of the study area. Orange arrows are from Barchi et al. (1991), yellow arrows are from Brozzetti and Lavecchia (1995), red arrows are orogen-orthogonal directions from this study, and green arrows are orogen-parallel directions from this study. The dashed blue line marks the morphological boundary between the Monti Martani Ridge and the Medio Tiberino and Terni Plio-Quaternary basins.
5.3 Paleostress evolution
Paleostress analyses on the acquired fault slip data revealed three distinct reduced extensional tensors, with a sub-horizontal σ3 oriented NE–SW, NNE–SSW, or NW–SE (Figs. 7 and 17). Despite the fact that field observations did not allow us to establish the relative chronology between the two first extensional phases, the latter (i.e., the NW–SE σ3) clearly postdates the first two. In fact, the extensional paleostress tensor with a sub-horizontal σ3 oriented NW–SE has been reconstructed in the Cesi quarry based on sub-horizontal slickenlines that postdate normal to oblique/normal slickenlines on NW–SE-striking polyphase fault surfaces. The same relative chronology between normal/oblique and sinistral strike-slip striae on ∼ N–S-striking faults has also previously been reported from other sites along the Martani Fault System (Bonini et al., 2003). Moreover, in the Viepri area, sub-vertical calcite veins formed under NW–SE-trending extension clearly postdate the formation of cataclasite related to normal faults formed under an NE–SW extensional regime (Fig. 8). Thus, compared to previous structural studies on the MMFS (Fig. 17), our work allowed us to better define the evolution of the local paleostress tensor as belonging to a transition from orogen-orthogonal extension (from NE–SW to NNE–SSW directions of extension) to a previously unknown local phase of orogen-parallel extension (NW–SE direction of extension).
The paleostress tensors with a sub-horizontal NE–SW and NNE–SSW σ3 do not necessarily relate to two distinct tectonic phases. In fact, as discussed above, numerical models have shown that, in the case of oblique rifting, with the regional extension direction oblique (∼ 45°) to inherited structures, the orientation of the local extensional stress tensor may evolve through time and pass from slightly oblique to the regional extension direction to almost perpendicular to the inherited structures (Brune, 2014, 2016). Thus, both local paleostress tensors might relate to the same phase of extension with a regional sub-horizontal minimum stress axis oriented NE–SW and compatible with the overall post-orogenic tectonic regime of the internal part of the northern and central Apennines (Mariucci and Montone, 2024).
Irrespective of whether this interpretation is correct, this mechanism cannot be used to explain the extensional paleostress tensor with a sub-horizontal σ3 oriented NW–SE. This paleostress tensor refers to an extensional tectonic event that postdates the formation of the main NW–SE-striking faults that developed under overall NE–SW extension. Moreover, all the measured faults are sutured by Upper Pleistocene deposits (Fig. 11, 13, and 15), and no fault has been observed to dislocate this latter sedimentary unit. Thus, the paleostress tensor constraining NW–SE extension is likely related to a short-lived tectonic pulse of orogen-parallel extension that took place at some point between the Early and the Late Pleistocene. The geodynamic causes of this orogen-parallel extensional event remain unclear. However, this event may possibly correlate chronologically with a regional phase of increased uplift rate and increased normal fault activity affecting the northern Apennines during the Early–Middle Pleistocene (Dramis, 1992).
The coexistence of orogen-parallel and orogen-orthogonal extensional structures has already been documented in several parts of the southern Apennines. There, some authors proposed that orogen-parallel extension preceded orogen-orthogonal extension and developed in response to the progressive thrust belt bending after the opening of the southern Tyrrhenian Sea during Early Miocene–Pliocene times (Oldow et al., 1993; Ferranti et al., 1996; Ferranti and Oldow, 1999). A similar interpretation cannot be applied to the orogen-parallel extensional event of our study because oroclinal bending in the internal Umbria–Marche portion of the northern Apennines that caused arcuation of the orogen occurred during the Late Miocene–Pliocene (Caricchi et al., 2014) and is thus older than the timing in which we frame the orogen-parallel extensional event in the MMR documented in this study. However, studies on recent seismic sequences along the N–S-trending boundary between the central and southern Apennines (Fig. 1a) have shown that orogen-parallel and orogen-orthogonal extension can locally coexist in an overall tectonic context of orogen-orthogonal extension (Milano et al., 2002, 2008). This geological context has much affinity with the tectonic setting of the Monti Martani area in terms of orientation of the post-orogenic extensional stress tensor and of the structural inheritance. In fact, they document seismic sequences along a structural inheritance oriented N–S and subject to an NE–SW-oriented post-orogenic extensional stress field. Similarly, the area of the Monti Martani is located in the vicinity of the Olevano–Antrodoco lineament, which represents the boundary between the northern and central Apennines (Parotto and Praturlon, 1975; Castellarin et al., 1978; Cosentino et al., 2010; Fig. 1a) and also represents a strong N–S-oriented structural inheritance and is subject to an NE–SW-oriented post-orogenic extensional stress field.
Another possible explanation for the orogen-parallel extensional event documented in this study is that it represents local perturbations of the regional stress field in relay zones between extensional faults in a mature stage of their evolution. In fact, in classical examples of release faults in extensional settings (e.g., Destro, 1995; Roberts, 1996; Destro et al., 2003), fault slip directions measured close to the main fault tips or along cross faults can differ by almost 90° from the regional direction of extension. Also, numerical models have shown that such perturbations of the regional stress field preferentially occur at the tips of isolated faults or in relay zones between overlapping faults (Kattenhorn et al., 2000). This mechanism can also account for the coexistence of orogen-orthogonal and orogen-parallel extensional seismicity in the internal part of the Apennines (as discussed earlier in this section) in the overall regional tectonic context of Quaternary orogen-orthogonal extension.
5.4 Active seismotectonics and fault displacement hazard
The distribution of active seismicity suggests that the MMR is a seismically active region that responds to the extensional tectonic regime currently shaping the internal domain of the Apennines. However, whether this ridge contains active and capable faults remains a matter of debate. This debate has practical implications on the estimate of fault displacement hazard and related seismic risk, since several settlements and civil infrastructures are built along and across the fault system.
The IAEA (2010) guidelines propose that, in interplate settings, a fault should be regarded as active and capable if it has produced permanent surface deformation in the Upper Pleistocene–Holocene time interval. Machette (2000) recommends that the reliable definition of the state of activity of a fault for seismic hazard assessment should consider a time interval that includes several earthquake cycles. By applying this concept to the extensional domain of the Apennines, Galadini et al. (2012) propose that a normal fault can be regarded as active and capable if it contains evidence of activation since the Middle Pleistocene, unless it is sealed by landforms or deposits older than the Last Glacial Maximum (i.e., ca. 20 ka). The guidelines of the Italian Department for Civil Protection consider a shorter time interval for the definition of active and capable faults, which should be considered such if they display evidence of surface rupture in the last 40 kyr (Technical Commission on Seismic Microzonation, 2015).
The ITHACA catalogue, which applies the definition of active and capable faults proposed by the IAEA (2010) guidelines, regards the Martani Fault System as active and capable by attributing a generic Pleistocene age to its activity (ITHACA Working Group, 2019). However, the supporting evidence for this statement is very dubious, since it is based on a large-scale neotectonic map of Italy (Ambrosetti et al., 1987b), which impacts its reliability. Based on the interpretation of geomorphic features such as tectonically displaced geomorphic markers, Bonini et al. (2003) suggested that some segments of the MMFS cut through Upper Pleistocene slope debris deposits and displace the Holocene topography, with reactivated N–NNW-striking fault segments showing oblique normal/sinistral kinematics and neoformed NW–SE-striking fault segments having normal kinematics, both affecting the Meso-Cenozoic carbonate bedrock. The same study supports these inferences by interpreting ruptures on the decumanus (i.e., a Roman road) at the archeological site of Carsulae as the result of surface faulting during a historical earthquake (around the fifth century CE). If these interpretations are correct, the MMFS should be regarded as active and capable. However, subsequent archeological and geophysical studies have confuted this hypothesis by showing that the surface deformation at the Carsulae site is related to the collapse of a doline in the Lower Pleistocene travertine during Roman times rather than to a seismic event (Aringoli et al., 2009; Bottari and Sepe, 2013; Bottari et al., 2017).
Our field observations document no faulting in deposits younger than the Lower Pleistocene. Where direct relationships between faults and the most recent sedimentary units were observed, faults always appear to be sutured by Upper Pleistocene sediments (Figs. 11, 13, and 15). This implies that the MMFS does not meet the requirements to be regarded as active and capable, and such a definition in the public catalogues should therefore be reconsidered. This is supported by the fact that the only unquestionable extensional faults cutting the Plio-Quaternary fill of the Medio Tiberino and Terni basins affect the Upper Pliocene (?)–Lower Pleistocene units (Brozzetti and Lavecchia, 1995; Brozzetti and Stoppa, 1995; Basilici, 1997; this study).
We have shown that the structural grain inherited from the Jurassic pre-orogenic rifting may still represent a major controlling factor in the tectonic evolution of this portion of the northern Apennines. In fact, although we did not document a reactivation of the western and southern boundaries of the Monti Martani paleo-horst, the present-day morphostructure of the study area seems to be controlled by the structural framework formed during the Early Jurassic rifting event. This structural inheritance also influenced the structural pattern of the Apennine orogeny in this sector. Most of the post-orogenic extensional faults that we have documented in this study are broadly orthogonal to the regional extension direction (i.e., NE–SW). By contrast, the inherited ∼ N–S and ∼ E–W trends of the pre-orogenic structural grain have a substantial obliquity (∼ 45°) to the regional post-orogenic direction of extension (Fig. 16). This might suggest that, for high obliquity between the inherited structures and the regional stress field, the activation of neoformed structures with an optimal orientation with respect to the stress field is mechanically preferred over the reactivation of non-optimally oriented pre-existing faults, as suggested by previous studies, even though the dominant morphostructural trends can still reflect the inherited structural template. This non-optimal orientation between the local structural grain and the far-field stress seems to also favor the formation of fault systems composed of short (a few kilometers long) second-order or en echelon faults rather than the formation of faults that are tens of kilometers long (Fig. 16). This might have important implications on seismic hazard estimates in this kind of context, since fault length is linearly correlated with the maximum potential magnitude of a seismogenic source.
This work also confirms the importance of field-based structural geology approaches to determine the surface displacement hazard related to complex active fault systems. In fact, a detailed and careful field analysis on single fault segments can confirm or disprove indications that can derive from larger-scale studies or geomorphological inferences, which often have a much lower resolution and/or more controversial interpretations. This also makes it possible to gain a better understanding of the evolution of the local stress field through time, when a long-lasting stable tectonic regime interacts with a non-optimally oriented inherited structural template.
Paleostress analysis was performed with the Win-Tensor program (Delvaux, 1993), which uses a refined version of the right dihedron method (Angelier and Mechler, 1977) and an iterative rotational optimization procedure (Delvaux and Sperner, 2003). The program is available at http://damiendelvaux.be/Tensor/WinTensor/win-tensor.html (Tensor Program, 2024).
The data presented in this article were independently collected from our original field surveys. These research data can be accessed on request to the corresponding author.
The supplement related to this article is available online at: https://doi.org/10.5194/se-15-1525-2024-supplement.
RA: conceptualization, fieldwork, data collection, data analysis, visualization, and writing (original draft and review and editing). SB: fieldwork, data collection, and writing (review and editing). GoV: data analysis, writing (review and editing), and funding acquisition. GaV: conceptualization, fieldwork, data analysis, writing (review and editing), and funding acquisition.
The contact author has declared that none of the authors has any competing interests.
Publisher's note: Copernicus Publications remains neutral with regard to jurisdictional claims made in the text, published maps, institutional affiliations, or any other geographical representation in this paper. While Copernicus Publications makes every effort to include appropriate place names, the final responsibility lies with the authors.
This work was supported by the PE3 RETURN Project (CUP J33C22002840002; Riccardo Asti, Gianluca Vignaroli). We thank Fabrizio Fioroni for providing access to the Grotta Eolia site in Cesi and for his kind and valuable assistance during the cave exploration. Valerio Chiaraluce is thanked for stimulating discussions in the archeological site of Carsulae. Simone Fabbi and Costantino Zuccari are thanked for helpful discussions about the pre-orogenic inheritance in the Apennine system. We thank Gianluca Benedetti, Massimo Comedini, Stefano Rodani, and Giulia Tartaglia for stimulating discussions in the field and for encouraging us throughout the realization of this study. We thank topic editor Stefano Tavani and executive editor Federico Rossetti for the editorial handling of this work. This article benefited from thoughtful comments and suggestions by Marco Mercuri and an anonymous reviewer.
This paper was edited by Federico Rossetti and reviewed by Marco Mercuri and one anonymous referee.
Accordi, G.: Il reticolo dei Monti Martani, B. Soc. Geol. Ital., 95, 3–26, 1966.
Alfonsini, L.: Wrench tectonic in Central Italy, a segment of the Sabina Fauls, B. Soc. Geol. Ital., 114, 414–421, 1995.
Alfonsini, L., Funiciello, R., Mattei, M., Girotti, O., Maiorani, A., Preite Martinez, M., Trudu, C., and Turi, B.: Structural and geochemical features of the Sabina strike-slip fault (Central Apennines), B. Soc. Geol. Ital., 110, 207–230, 1991.
Ambrosetti, P., Carboni, M. G., Conti, M. A., Costantini, A., Esu, D., Gandin, A., Girotti, O., Lazzarotto, A., Mazzanti, R., Nicosia, U., Parisi, G., and Sandrelli, F.: Evoluzione paleogeografica e tettonica dei bacini tosco-umbro-laziali nel Pliocene e nel Pleistocene inferiore, Memorie della Società Geologica Italiana, 19, 573–580, 1978.
Ambrosetti, P., Carboni, M. G., Conti, M. A., Esu, D., Girotti, O., La Monica, G. B., Landini, B., and Parisi, G.: Il Pliocene ed il Pleistocene inferiore del bacino del Fiume Tevere nell'Umbria meridionale: The Pliocene and the Lower Pleistocene of the Tevere Basin in Southern Umbria, Geogr. Fis. Din. Quat., 10, 10–33, 1987a.
Ambrosetti, P., Bosi, C., Carraro, F., Ciaranfi, N., Panizza, M., Papani, G., Vezzani, L., and Zanferrari, A.: Neotectonic Map of Italy: Scale 1:500000, Consiglio Nazionale delle Ricerche, Progetto Finalizzato Geodinamica, Sottoprogetto Neotettonica. Litografia Artistica Cartografica, Florence (Italy), 1987b.
Angelier, J. and Mechler, P.: Sur une méthode graphique de recherche des contraintes principales également utilisable en tectonique et en seismologie: la méthode des dièdres droits, B. Soc. Geol. Fr., 7-XIX, 1309–1318, 1977.
Aringoli, D., Farabollini, P., Gentili, B., Materazzi, M., and Pambianchi G.: Geomorphological evidences of natural disasters in the roman archaeological site of Carsulae (Tiber basin, Central Italy), in: Ol' man river: Geo-Archaelogical Aspects of Rivers and River plains, Ghent, edited by: de Dapper, M., Vermeulen, F., Deprez, S., and Taelman, D., Academia Press, 5–20, ISBN 9789038214047, 2009.
Autin, J., Bellahsen, N., Leroy, S., Husson, L., Beslier, M. O., and d'Acremont, E.: The role of structural inheritance in oblique rifting: Insights from analogue models and application to the Gulf of Aden, Tectonophysics, 607, 51–64, https://doi.org/10.1016/j.tecto.2013.05.041, 2013.
Barchi, M.: Una sezione geologica bilanciata attraverso il settore meridionale dell'Appennino umbro-marchigiano: l'Acquasparta-Spoleto-Accumuli, Studi Geologici Camerti, Vol. Spec. 1991/1, CROP 03, 347–362, 1991.
Barchi, M.: The Neogene–Quaternary evolution of the Northern Apennines: Crustal structure, style of deformation and seismicity, J. Virtual Explor, 36, 1–24, 2010.
Barchi, M., Brozzetti, F., and Lavecchia, G.: Analisi strutturale e geometria dei bacini della media valle del Tevere e della Valle Umbra, B. Soc. Geol. Ital., 110, 65–76, 1991.
Barchi, M., Carboni, F., Michele, M., Ercoli, M., Giorgetti, C., Porreca, M., Azzaro, S., and Chiaraluce, L.: The influence of subsurface geology on the distribution of earthquakes during the 2016–2017 Central Italy seismic sequence, Tectonophysics, 807, 228797, https://doi.org/10.1016/j.tecto.2021.228797, 2021.
Basilici, G.: II Bacino continentale Tiberino (Plio–Pleistocene, Umbria): analisi sedimentologica e stratigralica, PhD thesis, University of Bologna, 323 pp., 1992.
Basilici, G.: Evoluzione deposizionale del ramo sud-occidentale del Bacino Tiberino (Plio–Pleistocene, Umbria): da un sistema lacustre profondo as una piana alluvionale, Abstract for the conference: Le Conche Intermontane, 13–15 September 1993, Rome, 11–13, https://amq.aiqua.it/index.php/amq/article/view/1307 (last access: 20 December 2024), 1993.
Basilici, G.: Sedimentary facies in an extensional and deep-lacustrine depositional system: the Pliocene Tiberino Basin, Central Italy, Sediment. Geol., 109, 73–94, 1997.
Bernoulli, D., Kälin, O., and Patacca, E.: A sunken continental margin of the Mesozoic Tethys: The northern and central Apennines, in: Symposium: “Sédimentation Jurassique W européen”, 1977, Paris, Vol. 1, edited by: Beaudoin, B. and Purser, B. H., Publication Spécial Association Sédimentologique Française, 197–210, https://www.sedimentologie.fr/edition/la-sedimentation-du-jurassique-w-europeen/ (last access: 19 December 2024), 1979.
Berthé, D., Choukroune, P., and Jegouzo, P.: Orthogneiss, mylonite and non coaxial deformation of granites: the example of the South Armorican Shear Zone, J. Struct. Geol., 1, 31–42, 1979.
Boccaletti, M. and Guazzone, G.: Remnant arcs and marginal basins in the Cainozoic development of the Mediterranean, Nature, 252, 18–21, 1974.
Boccaletti, M., Elter, P., and Guazzone, G.: Plate tectonic models for the development of the Western Alps and Northern Apennines, Nature, 234, 108–111, 1971.
Boccaletti, M., Coli, M., Eva, C., Ferrari, G., Giglia, G., Lazzarotto, A., Merlanti, F., Nicolich, R., Papani, G., and Postpischl, D.: Considerations on the seismotectonics of the Northern Apennines, Tectonophysics, 117, 7–38, 1985.
Boncio, P., Brozzetti, F., and Lavecchia, G.: Architecture and seismotectonics of a regional low-angle normal fault zone in Central Italy, Tectonics, 19, 1038–1055, 2000.
Bonini, M.: Chronology of deformation and analogue modelling of the Plio–Pleistocene `Tiber Basin': implications for the evolution of the Northern Apennines (Italy), Tectonophysics, 285, 147–165, 1998.
Bonini, M., Tanini, C., Moratti, G., Piccardi, L., and Sani, F.: Geological and archaeological evidence of active faulting on the Martana Fault (Umbria–Marche Apennines, Italy) and its geodynamic implications, J. Quaternary Sci., 18, 695–708, 2003.
Bottari, C. and Sepe, V.: The role of earthquakes, landslides and climate changes in the abandonment of the Roman Carsulae site (Tevere basin e Central Italy), Quatern. Int., 308–309, 105–111, 2013.
Bottari, C., Aringoli, D., Carluccio, R., Castellano, C., Caracciolo, F. A., Gasperini, M., Materazzi, M., Nicolosi, I., Pambianchi, G., Pieruccini, P., and Sepe, V.: Geomorphological and geophysical investigations for the characterization of the Roman Carsulae site (Tiber basin, Central Italy), J. Appl. Geophys., 143, 74–85, https://doi.org/10.1016/j.jappgeo.2017.03.021, 2017.
Brozzetti, F. and Lavecchia, G.: Evoluzione del campo degli sforzi e storia deformativa nell'area dei Monti Martani (Umbria), B. Soc. Geol. Ital., 114, 155–176, 1995.
Brozzetti, F. and Stoppa, F.: Le piroclastiti medio-pleistoceniche di Massa Martana-Acquasparta (Umbria): caratteri strutturali e vulcanologici, Il Quaternario, 8, 95–110, 1995.
Brune, S.: Evolution of stress and fault patterns in oblique rift systems: 3-D numerical lithospheric-scale experiments from rift to breakup, Geochem. Geophy. Geosy., 15, 3392–3415, https://doi.org/10.1002/2014GC005446, 2014.
Brune, S.: Rifts and rifted margins: a review of geodynamic processes and natural hazards, in: Plate Boundaries and Natural Hazards, vol. 219, edited by: Duarte, J. C. and Schellart, W. P., Geophysical Monograph Series, Am. Geophys. Un., https://doi.org/10.1002/9781119054146.ch2, 2016.
Bruni, F., Calamita, F., Maranci, M., and Pierantoni, P. P.: Il controllo della tettonica giurassica sulla strutturazione neogenica dei Monti Martani meridionali (Preappennino umbro), Studi Geologici Camerti, Vol. Spec. 1995/1, 121–135, 1995.
Butler, H. R. W., Mazzoli, S., Corrado, S., Donatis, M. D., Bucci, D. D., Gambini, R., Naso, G., Nicolai, C., Scrocca, D., Shiner, P., and Zucconi, V.: Applying thick-skinned tectonic models to the Apennine thrust belt of Italy—Limitations and implications, in: Thrust tectonics and hydrocarbon systems, edited by: McClay, M. C. R., AAPG, https://doi.org/10.1306/m82813c34, 2004.
Butler, R. W. H., Tavarnelli, E., and Grasso, M.: Structural inheritance in mountain belts: an Alpine–Apennine perspective, J. Struct. Geol., 28, 1893–908, 2006.
Buttinelli, M., Pezzo, G., Valoroso, L., De Gori, P., and Chiarabba, C.: Tectonics inversions, fault segmentation, and triggering mechanisms in the central Apennines normal fault system: Insights from high-resolution velocity models, Tectonics, 37, 4135–4149, https://doi.org/10.1029/2018TC005053, 2018.
Calamita, F. and Pierantoni, P. P.: Structural setting of the Southern Martani Mountains (Umbria Apennines: Central Italy), Memorie della Società Geologica Italiana, 48, 549–557, 1994.
Calamita, F. and Pierantoni, P. P.: Modalità della strutturazione neogenica nell'Appennino Umbro-Sabino (Italia Centrale), Studi Geologici Camerti, Vol. Spec. 1995/1, 153–169, 1995.
Calamita, F., Cello, G., Centamore, E., Deiana, G., Micarelli, A., Paltrinieri, W., and Ridolfi, M.: Stile deformativo e cronologia della deformazione lungo tre sezioni bilanciate dall'Appennino umbro-marchigiano alla costa adriatica, Studi Geologici Camerti, Vol. Spec. 1991/1, 295–314, 1991.
Calamita, F., Satolli, S., Scisciani, V., Esestime, P., and Pace, P.: Contrasting styles of fault reactivation in curved orogenic belts: Examples from the central Apennines (Italy), Geol. Soc. Am. Bull., 123, 1097–1111, 2011.
Capotorti, F. and Muraro, C.: Post-rift extensional tectonics at the edge of a carbonate platform: insights from the Middle Jurassic–Early Cretaceous Monte Giano stratigraphic record (central Apennines, Italy), Geol. Acta, 19, 0012, 2021.
Capotorti, F. and Muraro, C.: Jurassic to Quaternary reactivation of inherited structures in the central Apennines, Ital. J. Geosci., 143, 37–59, 2024.
Cardello, G. L. and Doglioni, C.: From Mesozoic rifting to Apennine orogeny: The Gran Sasso range (Italy), Gondwana Res., 27, 1307–1334, https://doi.org/10.1016/j.gr.2014.09.009, 2015.
Caricchi, C., Cifelli, F., Sagnotti, L., Sani, F., Speranza, F., and Mattei M.: Paleomagnetic evidence for a post-Eocene 90° CCW rotation of internal Apennine units: A linkage with Corsica-Sardinia rotation?, Tectonics, 33, 374–392, https://doi.org/10.1002/2013TC003364, 2014.
Carminati, E., Lustrino, M., and Doglioni, C.: Geodynamic evolution of the central and western Mediterranean: Tectonics vs. igneous petrology constraints, Tectonophysics, 579, 173–192, 2012.
Castellarin, A., Colacicchi, R., and Praturlon, A.: Fasi distensive, trascorrenze e sovrascorrimenti lungo la 'linea Ancona-Anzio', dal Lias medio al Pliocene, Geologica Romana, 17, 161–189, 1978.
Cattuto, C., Gregori, L., Melelli, L., Taramelli, A., and Troiani, C.: Paleogeographic evolution of the Terni basin (Umbria, Italy), Proceedings of the Scientific Meeting `Geological and geodynamic evolution of the Apennines' in memory of Giampaolo Pialli, Foligno (PG), 16–17 February 2000, B. Soc. Geol. Ital., Special Volume 1, 865–872, 2002.
Cavinato, G. P. and De Celles, P. G.: Extensional basins in the tectonically bimodal central Apennines fold-thrust belt, Italy: response to corner flow above a subducting slab in retrograde motion, Geology, 27, 955–958, 1999.
Cello, G., Mazzoli, S., Tondi, E., and Turco, E.: Active tectonics in the Central Apennines and possible implications for seismic hazard analysis in peninsular Italy, Tectonophysics, 272, 43–68, 1997.
Centamore, E., Deiana, G., Micarelli, A., and Potetti, M.: Il Trias-Paleogene delle Marche, Studi Geologici Camerti, Volume speciale “La Geologia delle Marche”, 9–27, 1986.
Centamore, E., Di Manna, P., and Rossi, D.: Kinematic evolution of the Volsci Range: a new overview, B. Soc. Geol. Ital., 126, 159, 2007.
Chiarabba, C. and Amato, A.: Vp and Vp/Vs images in the Mw 6.0 Colfiorito fault region (central Italy): A contribution to the understanding of seismotectonic and seismogenic processes, J. Geophys. Res., 108, 2248, https://doi.org/10.1029/2001JB001665, 2003.
Cipriani, A. and Bottini, C.: Early Cretaceous tectonic rejuvenation of an Early Jurassic margin in the Central Apennines: The “Mt. Cosce Breccia”, Sediment. Geol., 387, 57–74, 2019a.
Cipriani, A. and Bottini, C.: Unconformities, neptunian dykes and mass-transport deposits as an evidence for Early Cretaceous syn-sedimentary tectonics: New insights from the Central Apennines, Ital. J. Geosci., 138, 333–354, 2019b.
Cipriani, A., Fabbi, S., Lathuilière, B., and Santantonio, M.: A reef coral in the condensed Maiolica facies on the Mt Nerone pelagic carbonate platform (Marche Apennines): The enigma of ancient pelagic deposits, Sediment. Geol., 385, 45–60, 2019.
Cipriani, A., Caratelli, M., and Santantonio, M.: Geological mapping reveals the role of Early Jurassic rift architecture in the dispersal of calciturbidites: New insights from the Central and Northern Apennines, Basin Res., 32, 1485–1509, https://doi.org/10.1111/bre.12438, 2020.
Coltorti, M. and Bosellini, A.: Sedimentazione e tettonica nel Giurassico della dorsale marchigiana, Studi Geologici Camerti, 6, 13–21, 1980.
Coltorti, M., Pierantoni, P. P., and Pieruccini, P.: I depositi fluvio-lacustri di Montebibico (Monti Martani meridionali) ed il loro significato nell'evoluzione tettonico-sedimentaria del Bacino Tiberino, Studi Geologici Camerti, Vol. Spec. 1995/1, 305–314, 1995.
Conti, M. A. and Girotti, O.: Il Villafranchiano del ”lago Tiberino”, ramo Sud occidentale. Schema stratigrafico e tettonico, Geologica Romana, 16, 67–80, 1977.
Conti, P., Cornamusini, G., Carmignani, L., Motti, A., Natali, N., Bettucci, C., Lavorini, G., Pirro, A., Pizziolo, M., and Daniele, G.: An outline of the geology of the Northern Apennines (Italy), with geological map at scale, Ital. J. Geosci., 139, 146–164, https://doi.org/10.3301/IJG.2019.25, 2020.
Cosentino, D., Cipollari, P., Marsili, P., and Scrocca, D.: Geology of the Central Apennines: A regional review, in: The Geology of Italy, edited by: Beltrando, M., Peccerillo, A., Mattei, M., Conticelli, S., and Doglioni, C., Journal of the Virtual Explorer (Electronic Edition), 36, paper 11, 2010.
Cowie, P. A., Roberts, G. P., Bull, J. M., and Visini, F.: Relationships between fault geometry, slip rate variability and earthquake recurrence in extensional settings, Geophys. J. Int., 189, 143–160, https://doi.org/10.1111/j.1365-246X.2012.05378.x, 2012.
Curzi, M., Aldega, L., Bernasconi, S. M., Berra, F., Billi, A., Boschi, C., Franchini, S., van der Lelij, R., Viola, G., and Carminati, E.: Architecture and evolution of an extensionally-inverted thrust (Mt. Tancia Thrust, Central Apennines): Geological, structural, geochemical, and K–Ar geochronological constraints, J. Struct. Geol., 136, 104059, https://doi.org/10.1016/j.jsg.2020.104059, 2020.
Curzi, M., Cipriani, A., Aldega, L., Billi, A., Carminati, E., van der Lelij, R., Vignaroli, G., and Viola, G.: Architecture and permeability structure of the Sibillini Mts. Thrust and influence upon recent, extension-related seismicity in the central Apennines (Italy) through fault-valve behavior, Geol. Soc. Am. Bull., 136, 3–26, 2024.
Decandia, F. A. and Tavarnelli, E.: Cronologia delle deformazioni nell'area di Spoleto (Umbria sud-orientale), Studi Geologici Camerti, Vol. Spec. 1991/1, 329–331, 1991.
Delvaux, D.: The TENSOR program for paleostress reconstruction: exemples from the east African and the Baikal rift zones, EUG VII Strasbourg, France, 4–8 April 1993, Abstract supplement N.1 to Terra Nova, 5, 216, 1993.
Delvaux, D. and Sperner, B.: Stress tensor inversion from fault kinematic indicators and focal mechanism data: the TENSOR program, in: New Insights into Structural Interpretation and Modelling, edited by: Nieuwland, D., Geological Society of London Special Publication, 212, 75–100, 2003.
Del Ventisette, C., Bonini, M., Maestrelli, D., Sani, F., Iavarone, E., and Montanari, D.: 3D-thrust fault pattern control on negative inversion: An analogue modelling perspective on central Italy, J. Struct. Geol., 143, 104254, https://doi.org/10.1016/j.jsg.2020.104254, 2021.
Destro, N.: Release fault: a variety of cross fault in linked extensional fault systems in the Sergipe-Alagoas basin, northeast Brazil, J. Struct. Geol., 17, 615–629, 1995.
Destro, N., Szatmari, P., Alkmim, F. F., Magnavita, L. P.: Release faults, associated structures, and their control on petroleum trends in the Recôncavo rift, northeast Brazil, AAPG Bull., 87,1123–1144. https://doi.org/10.1306/02200300156, 2003.
Di Domenica, A., Turtù, A., Satolli, S., and Calamita, F.: Relationships between thrusts and normal faults in curved belts: New insight in the inversion tectonics of the central-northern Apennines (Italy), J. Struct. Geol., 42, 104–117, https://doi.org/10.1016/j.jsg.2012.06.008, 2012.
Doglioni, C., Gueguen, E., Harabaglia, P., and Mongelli, F.: On the origin of west-directed subduction zones and applications to the western Mediterranean, in: The Mediterranean basins: Tertiary ex-tension within the Alpine orogen, edited by: Durand, B., Jolivet, L., Horvath, F., and Seranne, M., Geological Society of London Special Publication, 156, 541–561, 1999.
Dramis, F.: Il ruolo dei sollevementi tettonici a largo raggio nella genesi del rilievo appenninico, Studi Geologici Camerti, 1992/1, 9–15, 1992.
Elter, P.: L'ensemble ligure, B. Soc. Geol. Fr., 17, 984—997, 1975.
Faccenna, C., Mattei, M., Funiciello, D., and Jolivet, L.: Styles of back-arc extension in the Central Mediterranean, Terra Nova, 9, 126–130, https://doi.org/10.1046/j.1365-3121.1997.d01-12.x, 1997.
Faccenna, C., Becker, T. W., Lucente, F. P., Jolivet, L., and Rossetti, F.: History of subduction and back arc extension in the Central Mediterranean, Geophys. J. Int., 145(3), 809–820, https://doi.org/10.1046/j.0956-540x.2001.01435.x, 2001.
Farinacci, A., Malantrucco, G., Mariotti, N., and Nicosia, U.: Ammonitico Rosso facies in the framework of the Martani Mountains paleoenvironmental evolution during the Jurassic, in: Rosso Ammonitico Symposium Proceedings, 16–21 June 1980, Rome, edited by: Farinacci, A. and Elmi, S., Edizioni Tecnoscienza, Roma, 311–334, http://pi.lib.uchicago.edu/1001/cat/bib/722066 (last access: 19 Decembver 2024), 1981.
Ferranti, L. and Oldow, J. S.: History and tectonic implications of low-angle detachment faults and orogen parallel extension, Picentini Mountains, Southern Apennines fold and thrust belt, Italy, Tectonics, 18, 498–526, 1999.
Ferranti, L., Oldow, J. S., and Sacchi, M.: Pre-Quaternary orogen-parallel extension in the Southern Apennine belt, Italy, Tectonophysics, 260, 325–347, 1996.
Galadini, F. and Galli, P.: Active tectonics in the Central Apennines (Italy) – input data for seismic hazard assessment, Nat. Hazards, 22, 225–270, 2000.
Galadini, F., Falcucci, E., Galli, P., Giaccio, B., Gori, S., Messina, P., Moro, M., Saroli, M., Scardia, G., and Sposato, A.: Time intervals to assess active and capable faults for engineering practices in Italy, Eng. Geol., 139–140, 50–65, 2012.
Galderisi, A. and Galli, P.: Coulomb stress transfer between parallel faults. The case of Norcia and Mt Vettore normal faults (Italy, 2016 Mw 6.6 earthquake), Results in Geophysical Sciences, 1–4, 100003, https://doi.org/10.1016/j.ringps.2020.100003, 2020.
Galluzzo, F. and Santantonio, M.: The Sabina Plateau: a new element in the Mesozoic palaeogeography of Central Apennines, B. Soc. Geol. Ital., 1, 561–588, 2002.
Gasparini, C., Iannaccone, G., and Scarpa, R.: Fault-plane solutions and seismicity of the Italian peninsula, Tectonophysics, 117, 59–78, 1985.
Hodge, M. S., Venvik, G., Knies, J., van der Lelij, R., Schönenberger, J., Nordgulen, Ø., Brönner, M., Nasuti, A., and Viola, G.: Multiscalar 3D temporal structural characterisation of Smøla island, mid-Norwegian passive margin: an analogue for unravelling the tectonic history of offshore basement highs, Solid Earth, 15, 589–615, https://doi.org/10.5194/se-15-589-2024, 2024.
IAEA: Seismic hazards in site evaluation for nuclear installations, Safety Standards Series No. SSG-9, https://www-pub.iaea.org/MTCD/Publications/PDF/PUB1950_web.pdf (last access: 19 December 2024), 2010.
ISIDe Working Group: Italian Seismological Instrumental and Parametric Database (ISIDe), Istituto Nazionale di Geofisica e Vulcanologia (INGV), https://doi.org/10.13127/ISIDE, 2007.
ITHACA Working Group: ITHACA (ITaly HAzard from CApable faulting), A database of active capable faults of the Italian territory. Version December 2019, ISPRA Geological Survey of Italy, https://sgi.isprambiente.it/ithaca/viewer/index.html (last access: 19 December 2024), 2019.
Kattenhorn, S. A., Aydin, A., Pollard, D. D.: Joints at high angles to normal fault strike: an explanation using 3-D numerical models of fault-perturbed stress fields, J. Struct. Geol., 22, 1–23, 2000.
Keep, M. and McClay, K. R.: Analogue modelling of multiphase rift systems, Tectonophysics, 273, 239–270, 1997.
Keller, J. V. A., Minelli, G., and Pialli, G.: Anatomy of late orogenic extension: The Northern Apennines case, Tectonophysics, 238, 275–294, https://doi.org/10.1016/0040-1951(94)90060-4, 1994.
Laurenzi, M., Stoppa, F., and Villa, I.: Eventi ignei monogenici e depositi piroclastici nel distretto Ultra-Alcalino Umbro-Laziale (ULUD): revisione, aggiornamento e comparazione dei dati cronologici, Plinius, 12, 61–65, 1994.
Lavecchia, G., Boncio, P., Brozzetti, F., Stucchi, M., and Leschiutta, I.: New criteria for seismotectonic zoning in Central Italy: insights from the Umbria–Marche Apennines, Proceedings of the Scientific Meeting `Geological and geodynamic evolution of the Apennines' in memory of Giampaolo Pialli, Foligno (PG), 16–17 February 2000, B. Soc. Geol. Ital., Special Volume 1, 881–890, 2002.
Machette, M. N.: Active, capable, and potentially active faults – a paleoseismic perspective, J. Geodyn., 29, 387–392, 2000.
Malinverno, A. and Ryan, W. B. F.: Extension in the Tyrrhenian sea and shortening in the Apennines as results of arc migration driven by sinking of the lithosphere, Tectonics, 5, 227–245, 1986.
Mantovani, E., Babbucci, D., Tamburelli, C., and Viti, M.: A review on the driving mechanism of the Tyrrhenian-Apennines system: implications for the present seismotectonic setting in the Central-Northern Apennines, Tectonophysics, 476, 22–40, https://doi.org/10.1016/j.tecto.2008.10.032, 2009.
Mariotti, N., Nicosia, U., Pallini, G., and Schiavinotto, F.: Kimmeridgiano recifale presso Case Canepine (M. Martani, Umbria): ipotesi paleogeografiche, Geologica Romana, 18, 295–315, 1979.
Mariucci, M. T. and Montone, P.: IPSI 1.6, Database of Italian Present-day Stress Indicators, Istituto Nazionale di Geofisica e Vulcanologia (INGV), https://doi.org/10.13127/IPSI.1.6, 2024.
Mariucci, M. T., Amato, A., and Montone, P.: Recent tectonic evolution and present stress in the Northern Apennines, Tectonics, 18, 108–118, 1999.
Mattila, J. and Viola, G.: New constraints on 1.7 Gyr of brittle tectonic evolution in southwestern Finland derived from a structural study at the site of a potential nuclear waste repository (Olkiluoto Island), J. Struct. Geol., 67, 50–74, 2014.
McClay, K. R. and White, M. J.: Analogue modelling of orthogonal and oblique rifting, Mar. Petrol. Geol., 12, 137–151, https://doi.org/10.1016/0264-8172(95)92835-K, 1995.
Mercuri, M., Tavani, S., Fabbi, S., Lavosi, G., and Carminati, E.: Long live the fault! Double inversion of a Mesozoic rift-related fault system in the central Apennines, Terra Nova, 36, 358–368, https://doi.org/10.1111/ter.12718, 2024.
Milano, G., Ventura, G., and Di Giovambattista, R.: Seismic evidence of longitudinal extension in the Southern Apennines chain (Italy): the 1997–1998 Sannio–Matese seismic sequence, Geophys. Res. Lett., 29, 65-1–65-4, https://doi.org/10.1029/2002GL015188, 2002.
Milano, G., Di Giovambattista, R., and Ventura, G.: Seismic activity in the transition zone between Southern and Central Apennines (Italy): evidences of longitudinal extension inside the Ortona-Roccamonfina tectonic line, Tectonophysics, 457, 102–110, 2008.
Mildon, Z. K., Roberts, G. P., Faure Walker, J. P., and Iezzi, F.: Coulomb stress transfer and fault interaction over millennia on non-planar active normal faults: the Mw 6.5–5.0 seismic sequence of 2016–2017, central Italy, Geophys. J. Int., 210, 1206–1218, 2017.
Molli, G.: Northern Apennine–Corsica orogenic system: an updated overview, Geological Society of London Special Publication,, 298, 413–442, 2008.
Montone, P., Mariucci, M. T., and Pierdominici, S.: The Italian present-day stress map, Geophys. J. Int., 189, 705–716, https://doi.org/10.1111/j.1365-246X.2012.05391.x, 2012.
Oldow, J. S., D'Argenio, B., Ferranti, L., Pappone, G., Marsella, E., and Sacchi, M.: Largescale longitudinal extension in the southern Apennines contractional belt, Italy, Geology, 21, 1123–1126, 1993.
Pace, P. and Calamita, F.: Push-up inversion structures vs. fault-bend reactivation anticlines along oblique thrust ramps: examples from the Apennines fold-and-thrust belt (Italy), J. Geol. Soc. London, 171, 227–238, https://doi.org/10.1144/jgs2013-053, 2014.
Parotto, M. and Praturlon, A.: Geological summary of the Central Apennines, Quaderni de 'La Ricerca Scientifica', 90, 257–311, 1975.
Patacca, E., Scandone, P., Di Luzio, E., Cavinato, G. P., and Parotto, M.: Structural architecture of the central Apennines: Interpretation of the CROP 11 seismic profile from the Adriatic coast to the orographic divide, Tectonics, 27, TC3006, https://doi.org/10.1029/2005TC001917, 2008.
Piccardi, L., Sani, F., Bonini, M., Boccaletti, M., Moratti, G., and Gualtierotti, A.: Deformazioni quaternarie nell'Appennino centro-settentrionale: evidenze ed implicazioni, Proceedings of the A. I. Q.UA. Congress `Tettonica quaternaria del territorio italiano: conoscenze, problemi ed applicazioni', Parma, 25–27 February 1997, Il Quaternario, 10, 273–280, 1997.
Pizzi, A. and Galadini, F.: Pre-existing cross-structures and active fault segmentation in the northern-central Apennines (Italy), Tectonophysics, 476, 304–319, 2009.
Pondrelli, S., Salimbeni, S., Ekström, G., Morelli, A., Gasperini, P., and Vannucci, G.: The Italian CMT dataset from 1977 to the present, Phys. Earth Planet. In., 159, 286–303, https://doi.org/10.1016/j.pepi.2006.07.008, 2006.
Principi, G. and Treves, B.: Il sistema corso-appenninico come prisma d'accrezione. Riflessi sul problema generale del limite Alpi–Appennini, Memorie della Società Geologica Italiana, 28, 549–576, 1984.
Regione Umbria – Servizio Geologico: Carta geologica dell'Umbria, https://dati.regione.umbria.it/dataset/carta-geologica-dell-umbria (last access: 19 December 2024), 2013.
Roberts, G. P.: Variation in fault-slip directions along active and segmented normal fault systems, J. Struct. Geol., 18, 835–845, 1996.
Rosenbaum, G. and Lister, G. S.: Neogene and Quaternary rollback evolution of theTyrrhenian Sea, the Apennines, and the Sicilian Maghrebides, Tectonics, 23, TC1013, https://doi.org/10.1029/2003TC001518, 2004.
Rosenbaum, G., Lister, G. S., and Duboz, C.: The Mesozoic and Cenozoic motion of Adria (central Mediterranean): constraints and limitations, Geodin. Acta, 17, 125–139, 2004.
Rovida, A., Locati, M., Camassi, R., Lolli, B., and Gasperini, P.: The Italian earthquake catalogue CPTI15, B. Earthq. Eng., 18, 2953–2984, https://doi.org/10.1007/s10518-020-00818-y, 2020.
Rovida, A., Locati, M., Camassi, R., Lolli, B., Gasperini, P., and Antonucci A.: Catalogo Parametrico dei Terremoti Italiani (CPTI15), versione 4.0, Istituto Nazionale di Geofisica e Vulcanologia (INGV), https://doi.org/10.13127/CPTI/CPTI15.4, 2022.
Sabbatino, M., Tavani, S., Vitale, S., Ogata, K., Corradetti, A., Consorti, L., Arienzo, I., Cipriani, A., and Parente, M.: Forebulge migration in the foreland basin system of the central-southern Apennine fold-thrust belt (Italy): New high-resolution Sr-isotope dating constraints, Basin Res., 33, 2817–2836, 2021.
Salvini, F., Billi, A., and Wise, D. U.: Strike-slip fault-propagation cleavage in carbonate rocks: the Mattinata fault zone, Southern Apennines, Italy, J. Struct. Geol. 21, 1731–1749, 1999.
Santantonio, M.: Facies associations and evolution of pelagic carbonate platform/basin systems: examples from the Italian Jurassic, Sedimentology, 40, 1039–1067, 1993.
Santantonio, M. and Carminati, E.: The Jurassic rifting evolution of the Apennines and Southern Alps (Italy): Parallels and differences, Geol. Soc. Am. Bull., 123, 468–484, 2011.
Santantonio, M., Cipriani, A., Fabbi, S., Citton, P., and Romano, M.: PCP-basin settings in the fossil record: state of the art – Guest editorial, Ital. J. Geosci., 139, 5–7, 2020.
Scheiber, T. and Viola, G.: Complex Bedrock Fracture Patterns: A Multipronged Approach to Resolve Their Evolution in Space and Time, Tectonics, 37, 1030–1062, https://doi.org/10.1002/2017TC004763, 2018.
Scisciani, V.: Styles of positive inversion tectonics in the central Apennines and in the Adriatic foreland: Implications for the evolution of the Apennine chain (Italy), J. Struct. Geol., 31, 1276–1294, https://doi.org/10.1016/j.jsg.2009.02.004, 2009.
Scisciani, V., Agostini, S., Calamita, F., Pace, P., Cilli, A., Giori, I., and Paltrinieri, W.: Positive inversion tectonics in foreland fold-and-thrust belts: A reappraisal of the Umbria–Marche Northern Apennines (Central Italy) by integrating geological and geophysical data, Tectonophysics, 637, 218–237, 2014.
Scognamiglio, L., Tinti, E., and Quintiliani, M.: Time Domain Moment Tensor (TDMT) [Data set], Istituto Nazionale di Geofisica e Vulcanologia (INGV), https://doi.org/10.13127/TDMT, 2006.
Tavani, S., Granado, P., Corradetti, A., Camanni, G., Vignaroli, G., Manatschal, G., Mazzoli, S., Muñoz, J. A., and Parente, M.: Rift inheritance controls the switch from thin-to thick-skinned thrusting and basal décollement re-localization at the subduction-to-collision transition, Geol. Soc. Am. Bull., 133, 2157–2170, 2021.
Tavani, S., Smeraglia, L., Fabbi, S., Aldega, L., Sabbatino, M., Cardello, G. L., Maresca, A., Schirripa Spagnolo, G., Kylander-Clark, A., Billi, A., Bernasconi, S. M., and Carminati, E.: Timing, thrusting mode, and negative inversion along the Circeo thrust, Apennines, Italy: How the accretion-to-Extension transition operated during slab rollback, Tectonics, 42, e2022TC007679, https://doi.org/10.1029/2022TC007679, 2023.
Tavarnelli, E.: The effects of pre-existing normal faults on thrust ramp development: an example from the Northern Apennines, Italy, Geol. Rundsch., 85, 363–371, 1996.
Tavarnelli, E., Butler, R. W. H., Decandia, F. A., Calamita, F., Grasso, M., Alvarez, W., and Renda, P.: Implications of fault reactivation and structural inheritance in the Cenozoic tectonic evolution of Italy, Geology of Italy, 1, 209–222, 2004.
Tavarnelli, E., Scisciani, V., Patruno, S., Calamita, F., Pace, P., and Iacopini, D.: The role of structural inheritance in the evolution of fold-and-thrust belts: insights from the Umbria–Marche Apennines, Italy, in: 250 Million Years of Earth History in Central Italy: Celebrating 25 Years of the Geological Observatory of Coldigioco, edited by: Koeberl, C. and Bice, D. M., Geological Society of America, Boulder, CO, Special Paper no. 542, pp. 191–212. 2019.
Technical Commission on Seismic Microzonation: Land Use Guidelines for Areas with Active and Capable Faults (ACF), Conference of the Italian Regions and Autonomous Provinces, Civil Protection Department, Rome, 1–26, https://www.centromicrozonazionesismica.it/documents/18/GuidelinesForSeismicMicrozonation.pdf (last access: 19 December 2024), 2015.
Tensor Program: Fault-kinematic analysis and tectonic stress tensor inversion, http://damiendelvaux.be/Tensor/WinTensor/win-tensor.html (last access: 19 December 2024), 2024.
Vai, G. B. and Martini, I. P.: Anatomy of an Orogen: The Apennines and Adjacent Mediterranean Basins, Springer, Dordrecht, 633 pp., https://doi.org/10.1007/978-94-015-9829-3, 2001.
Vezzani, L., Festa, A., and Ghisetti, F.: Geology and Tectonic Evolution of the Central-Southern Apennines, Italy, Special paper 469, Geological Society of America, Boulder, CO, USA, https://doi.org/10.1130/2010.2469, 2010.
Viola, G., Venvik Ganerød, G., and Wahlgren, C.-H.: Unravelling 1.5 Gyr of brittle deformation history in the Laxemar-Simpevarp area, SE Sweden: a contribution to the Swedish site investigation study for the disposal of highly radioactive nuclear waste, Tectonics, 28, TC5007, https://doi.org/10.1029/2009TC002461, 2009.
Viola, G., Kounov, A., Andreoli, M. A. G., amd Mattila, J.: Brittle tectonic evolution along the western margin of South Africa: more than 500 Myr of continued reactivation, Tectonophysics, 514–517, 93–114, 2012.
Viola, G., Musumeci, G., Mazzarini, F., Tavazzani, L., Curzi, M., Torgersen, E., van der Lelij, R., and Aldega, L.: Structural characterization and K–Ar illite dating of reactivated, complex and heterogeneous fault zones: lessons from the Zuccale Fault, Northern Apennines, Solid Earth, 13, 1327–1351, https://doi.org/10.5194/se-13-1327-2022, 2022.
Wang, L., Maestrelli, D., Corti, G., Zou, Y., and Shen, C.: Normal fault reactivation during multiphase extension: analogue models and application to the Turkana depression, East Africa, Tectonophysics, 811, 228870, https://doi.org/10.1016/j.tecto.2021.228870, 2021.
Wells, D. L. and Coppersmith, K. J.: Empirical relationships among magnitude, rupture length, rupture area, and surface displacement, B. Seismol. Soc. Am., 84, 974–1002, https://doi.org/10.1785/BSSA0840040974, 1994.
Ziegler, P. A.: Evolution of the Arctic-North Atlantic and the Western Tethys, AAPG Memoir., 43, 1–198, 1988.
Zuccari, C., Viola, G., Curzi, M., Aldega, L., and Vignaroli, G.: What steers the “folding to faulting” transition in carbonate-dominated seismic fold-and-thrust belts? New insights from the Eastern Southern Alps (Northern Italy), J. Struct. Geol., 157, 104560, https://doi.org/10.1016/j.jsg.2022.104560, 2022.