the Creative Commons Attribution 4.0 License.
the Creative Commons Attribution 4.0 License.
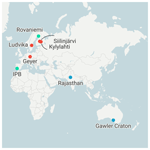
Preface: State of the art in mineral exploration
Ramon Carbonell
Solveig Pospiech
Liam A. Bullock
Fernando Tornos
- Article
(857 KB) - Full-text XML
- BibTeX
- EndNote
Minerals that comprise raw materials for energy, metal, construction and other industrial applications are considered strategic commodities, fundamental in stock markets worldwide, and key ingredients to sustain our ever more technology-based society (Wellmer et al., 2019). The utilization of such economically important minerals has shown a continued steady increase since the early twentieth century, with a greater focus in recent years on resources required for the development of renewable technologies, such as wind and solar operations, and for electrification of domestic and transportation systems (e.g. concrete, aluminium, chromium, copper, iron, manganese, molybdenum, nickel, zinc or rare earths) (Meinert et al., 2016). As our society ramps up the global transition to low-carbon energies and a reduced reliance on fossil fuels, the inevitable rise in consumption and demand for a more diverse range of resources can only be facilitated through increasingly novel methods of mineral exploration (Ali et al., 2017).
The aim of mineral exploration has been the same since the dawns of early civilization: to uncover evidence for mineral prospects in the ground for sale or use by means of a range of activities. Primitive approaches of mineral exploration and sourcing of minerals include basic methods of prospecting, such as gold panning, which has been practiced for several millennia, and surface scraping with tools made from stone, bone or volcanic glass at sites where metal-rich boulders were found at the surface (Villalobos-García and Odriozola-Lloret, 2016). Modern approaches, consolidated during the twentieth century, include geophysical surveying, bedrock mapping, geochemical sampling and diamond drilling (Marjoribanks, 2010). While many of these methods still form the basis for exploratory reconnaissance campaigns, the time and financial costs, the impact on both the land and available water resources and reliance on fossil fuels remain an issue for prospectors and mining stakeholders. Furthermore, the continual exhaustion of easily accessible major ore deposits, the increasing demand for major industrial methods – and ever more important environmental technology (E-tech) critical raw metals – and, for some countries, the need for a lower reliance on imported supplies for environmental as well as political reasons means there is a greater need for new, cheaper, sustainable and higher resolution methods of mineral exploration (Alcalde et al., 2020; Malehmir et al., 2020).
To meet the growing volume of mineral resources required to sustain our technological economy, the mining industry has strongly increased its mineral exploration efforts in the last two decades. The research community is participating in these efforts, as demonstrated by the growing body of literature devoted to mineral exploration in its various forms (Fig. 1). While conventional methods still dominate the market, novel approaches or methods adopted from the oil and gas industry are gaining acceptance in mineral exploration. The use of advanced geophysical techniques (Malehmir et al., 2012; Dentith et al., 2018), geochemical big data analysis (Zuo and Xiong, 2018; Yousefi et al., 2019), high resolution remote sensing tools (Kirsch et al., 2018; Adiri et al., 2020), or even uncrewed robotic devices (Lopes et al., 2020), are revolutionising the search for resources worldwide. These advances have spurred the launch of multiple research projects in the last decade, aimed to develop novel exploration tools and methodologies.
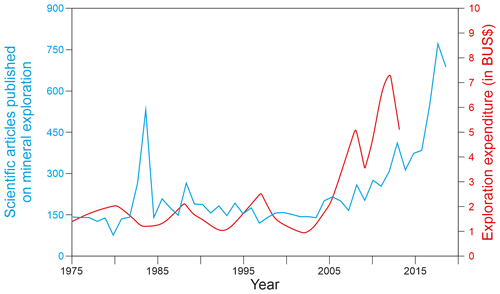
Figure 1Number of scientific articles published on the topic of mineral exploration (in blue, source: Scopus) and global expenditure in mineral exploration (in red, source: Schodde, 2014) since 1975.
This special issue is intended to bring together new insights into the state of the art in mineral exploration using a variety of developing or evolving techniques, including passive and active seismic tomography, magnetic and multispectral remote sensing, and geochemical vector, alteration, and anomaly mapping applications. As well as a broad disciplinary focus, the special issue also includes a wide geographic analysis, with studies spread across four continents (Fig. 2). The scientific contributions assembled here provide a detailed assessment of the current state of exploration and an indication of the direction in which mineral exploration is headed in the remaining years of the 21st century.
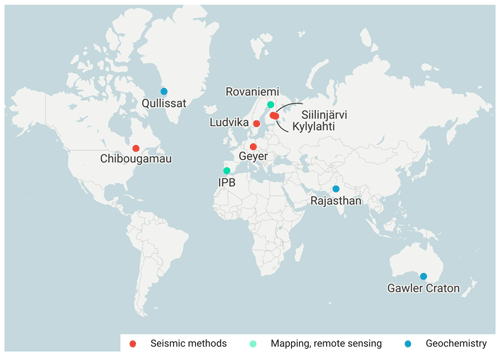
Figure 2Locations of the studies included in this special issue. Map created with Datawrapper (https://www.datawrapper.de/, last access: 27 July 2022).
This special issue comprises 13 articles broadly covering three major exploration methods: seismic, mapping and remote sensing, and geochemical-related methods (Fig. 2, Table 1). These methods were applied in a wide range of mineral settings in four different continents, highlighting their broad applicability.
In this preface we give a brief summary how the manuscripts contribute to this Special Issue along their methodical background.
Table 1Methods used, mineral targets and location of the mine prospects of the 13 articles compiled in this special issue.
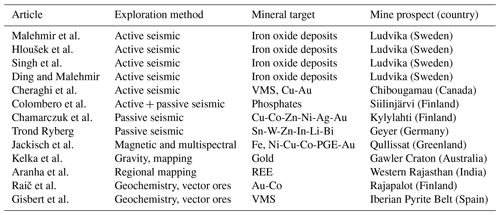
2.1 Seismic methods
Like many other exploration techniques, seismic imaging (seismic reflection in particular) applications were developed for the oil and gas industry, and their uses in resource exploration have been conventionally limited to the exploration for hydrocarbons or other types of reservoirs (Alcalde et al., 2013, 2021; Ikelle and Amundsen, 2018; Sena-Lozoya et al., 2020). However, the advances in data acquisition and processing achieved in the last two decades have improved their resolution power and reduced costs, making such approaches more affordable and able to address the challenges related to mineral exploration (Eaton et al., 2003; Malehmir et al., 2012; Bellefleur et al., 2019; Martinez et al., 2020; Gil et al., 2021). Passive seismic exploration using ambient seismic noise is also creating a revolution in seismology, mainly thanks to its versatility and reduced cost compared to conventional active exploration (Boullenger et al., 2015; Romero and Schimmel, 2018; Sánchez-Pastor et al., 2019). While the widespread implementation of seismic methods in mineral exploration is still in progress, there is a growing interest in the mining industry in the use of seismic methods.
Eight out of the 13 articles included in this special issue employ seismic methods for the exploration of mineral resources. Of these eight, four contributions report seismic studies carried out for the exploration of the Ludvika Mines (Bergslagen mineral district, central Sweden), as part of the European Commission's Horizon 2020-funded project “Smart Exploration”. The first article, by Malehmir et al. (2021) presents a sparse 3D seismic reflection dataset acquired in the Blötberget mining area, aimed to characterise the iron oxide deposits and their host rocks at depth. The ∼6 km2 3D survey utilised a 32 t vibrator truck to produce over 1000 shot points recorded in 1266 seismic recorders. In spite of the challenges related to the ground conditions and access, resulting in significant surface wave content, the processing applied allowed obtaining a good 3D image of the two main faults that determine the structural configuration of the Blötberget mining area. This work provides the first 3D image of the Blötberget mining area, and paves the way to other more advanced processing studies, also collected in this special issue (see Hloušek et al., 2022; Singh et al., 2022 below). This study highlights the potential of traditional active seismic methods to image subsurface structures in challenging crystalline environments.
In a following study, Hloušek et al. (2022) apply two pre-stack depth migration approaches to the 3D seismic dataset acquired at the Blötberget iron oxide mining site described in Malehmir et al. (2021). The authors apply a Kirchhoff pre-stack depth migration and Fresnel volume migration to the data, to produce a well-resolved 3D depth image of the deposit and its host rock. The Fresnel volume migration produced significantly better imaging results, and allowed to distinguish reflections linked to known mineralisation, providing a means of projection beyond the previously known mineralisation extents inferred from borehole data. The imaging results, verified by comparison to mapped mineralisation zones, will serve as the basis for further investigations, drillings and follow-up mine planning at the Blötberget mining site, and similar sites worldwide.
The third contribution from the Ludvika mining area, by Singh et al. (2022), presents the implementation of a time-domain 3D acoustic full-waveform inversion scheme to the same sparse 3D dataset. Within this approach, the authors used 216 shots to produce an accurate velocity model of the subsurface of the target area. The obtained velocity model provides higher resolution and reaches greater depth than the one obtained using conventional first arrival travel-time tomography, making it very suitable for pre-stack depth imaging. The velocity model is thus used pre-stack depth with reverse time migration to produce a 3D image of the study area, in which the authors are able to map the mineralization and explore its extent at ca. 1000 m depth. The presented approach successfully combines full-waveform inversion with pre-stack depth imaging with reverse time migration, and highlights the use of more complex processing techniques to the seismic imaging of challenging subsurface environments, such mineral prospects in crystalline settings.
In the last contribution to the imaging of the Ludvika Mines, Ding and Malehmir (2021) investigate the potential of reverse time migration to image the deep (1 km) subsurface in hard rock environments. To test this technique the authors apply reverse time migration to a 2.2 km long 2D section acquired in 2016 (Markovic et al., 2020), employing 451 seismic receivers and a 500 kg drop hammer as source. The authors applied a pre-processing workflow to enhance the signal-to-noise ratio and improve the reverse time migrated image. The seismic section obtained allows identifying the mineralisation-bearing horizons down to 1200 m, and provides an improved image of the intersection between the mineralised horizons and the two major faults that control the structure in the area.
On the other side of the Atlantic, Cheraghi et al. (2021) apply pre- and post-stack migration algorithms to two high-resolution seismic profiles in the Abitibi subprovince (Canada), targeting volcanogenic massive sulphide (VMS) and hydrothermal Cu-Au deposits. The two high resolution profiles are located on top of regional line acquired as part of the Metal Earth project (Naghizadeh et al., 2019). The authors apply dip moveout corrections (DMO) and pre-stack time migration (PSTM) algorithms to obtain images of the main faults in the area, as well as identifying two diffraction sets that could be targeted in future exploration efforts. In their analysis, Cheraghi et al (2021) confirm that both techniques, DMO and PSTM, are very sensitive to irregularities in the acquisition geometry, in this case produced by the crooked footprint of the profiles as well as gaps of shots or receivers during the acquisition. This is, unfortunately, a common challenge in land seismic exploration, which can lead to misinterpretation of out-of-plane reflections.
Passive seismic data using ambient seismic noise is also receiving increased interest from the mineral exploration community (Cheraghi et al., 2015; Da Col et al., 2020; Xie et al., 2021), mainly thanks to its reduced cost and easy implementation, compared to active seismic surveying. In their contribution, Colombero et al. (2022) combine active and passive data to produce S-wave velocity (Vs) models of the Siilinjärvi phosphorus mine in Finland. The authors present a workflow for the semi-automatic picking of dispersion curves from ambient seismic noise, which helped to reduce significantly the time and computational costs associated with this otherwise burdensome task. The dispersion curve results of the passive data are comparable to those of the active data for the overlapping frequencies analysed, which helped the authors to combine the two datasets with confidence. The passive data provides information from deeper portions of the subsurface, allowing to extend the Vs models to greater depth. The final Vs model has allowed the authors to follow the trace of the mineralised deposits and of the complex set of intruding dikes.
In their contribution, Chamarczuk et al. (2022) present a novel imaging experiment using passive seismic data in the Kylylahti polymetallic mine, also in Finland. The authors follow the track opened by Cheraghi et al. (2015) to produce a 3D seismic virtual-source survey using ambient noise seismic interferometry, applied in this case to a hard rock environment. The authors use 30 d of ambient noise record to generate almost 1000 virtual-source gathers, forming a full 3D virtual-source survey that can then be processed using a conventional processing workflow for active data. The seismic volume generated from the passive dataset is comparable with that obtained from an active dataset acquired in the same area. This comparison validates the usefulness of virtual-source surveys to image the subsurface, and confirms that active mine environments provide ambient noise suitable for imaging purposes.
In the last seismic exploration study compiled in this special issue, Ryberg et al. (2022) carry out an ambient seismic noise tomographic survey to derive the 3D shear wave velocity model of the subsurface of the Geyer-Ehrenfriedersdorf mining district (Germany). This area hosts world-class magmatic hydrothermal ore deposits associated with the emplacement of the Geyer granite, containing important commodities such as Sn, W, In, Cu, Co, Bi, Sb and Au. The authors use data from 400 short period seismic stations to derive a 3D shear wave velocity model of the subsurface using tomographic inversion based on Bayesian statistics. The seismic data acquired is complemented with an airborne electromagnetic dataset. Both the obtained velocity and the resistivity data allow identifying the orientation of the main subsurface structures, but their exploration potential is boosted when combined. The authors use an unsupervised classification method to obtain nine different rock classes based on their velocity and resistivity, and validated them with drill core data. The methodology presented by the authors allows overcoming an important challenge that commonly affects mineral exploration in crystalline settings, which is the lack of contrasting petrophysical properties of the target rocks. This study provides an excellent example of sustainable exploration, combining successfully the two low-impact, cost-effective geophysical methods to obtain a valuable model of the prospect area.
2.2 Remote sensing methods
Mining areas can be challenging targets for exploration due to the lack accessibility to the resources, for example, due to rugged topography or poor exposure of relevant geological formations. This is the case in the West Greenland flood basalt province, which hosts rare native iron occurrences and Ni-Cu-Co-PGE-Au mineralizations. To overcome those challenging conditions, Jackisch et al. (2022) acquired high-resolution magnetic and multispectral remote sensing data using drones in a 6×3 km survey in the Qullissat area, northern Disko Island. This area is characterized by a rough topography (600–900 m a.s.l.) and contains debris affected by mass-movement broadly covered by vegetation, therefore showing limited outcrops. The drone data acquired allow the authors to link topography, surface mineralogy and magnetic data to provide both direct and indirect information about potential sulfide-enriched targets. The acquired magnetic data is combined with the multispectral data, which bands are sensitive for the detection of iron-related spectral features, and with the petrophysical properties obtained from available drill-core data. With all these elements, the authors apply a 3D vector inversion model to obtain an estimation of the magnetic properties and to constrain the shape and distribution of the mineralization body. In spite of the accessibility and topography issues, and with a target mineralization body mainly buried, the authors present a workflow that allows to precisely map the target body at depth. This study demonstrates the potential of unoccupied aerial system (UAS) exploration surveys in challenging and remote areas.
Mineral deposits are often found associated to surface lineaments related to geological structures, such as faults. However, sometimes those lineaments are difficult to detect or interpret due to limited surface outcrops available in the area. Geophysical methods have been extensively employed in the past to detect these “hidden” lineaments, but the lineaments are often not well-correlated with surface expressions. Kelka et al. (2022) present a workflow to automatically extract and analyse lineaments by combining surface (digital elevation models and radiometric) and subsurface (total magnetic intensity and gravity) datasets. To test their workflow, they focused on the Olympic iron oxide copper gold province, at the eastern margin of the Gawler Craton, South Australia, where the Tarcoola mine is located. The Tarcoola mine area hosts disseminated Au mineralization in brittle to brittle-ductile faults and shear zones. The authors propose the combination of geophysical lineaments derived by automatic gradient extraction together with either manually or automatically mapped surface lineaments. From the analysis of the lineaments, the authors observe that the E–W directions dominate in both the surface and subsurface datasets. In their study, they also find that manual interpretation is suitable to interpret region-scale trends, while the automatically extracted features represents smaller-scale, locally relevant structures. The combination of surface and subsurface lineaments is thus an effective method to produce targeting maps for mineral exploration.
Regional targeting is one of the first stages of the mineral exploration workflow. One of the most common regional exploration methods, mineral prospectivity modelling, involves the combination of different data (e.g. geological, geophysical, geochemical, structural, remote sensing) targeted to a specific deposit type to develop “predictor maps”. In their contribution, Aranha et al. (2022) feed a conceptual model of rare earth elements (REE) associated with carbonatite–alkaline complexes in western Rajasthan (India) by combining nine layers of input data to generate predictor maps. Then, a knowledge-driven artificial intelligence technique (fuzzy inference system) is used in combination with uncertainty modelling to produce maps that include information on the fertility sources and geodynamic setting, lithospheric transportation architecture and near-surface emplacement. These maps are finally combined into a map of prospective REE targets, which can be then subject of higher resolution studies to constrain the targets (e.g. geophysics, field mapping etc). The prospectivity analysis methodology presented in this contribution can also be implemented in other regions of the world with similar geodynamic settings.
2.3 Geochemical methods
The study conducted by Raič et al. (2022) demonstrates the use of geochemical techniques and vector determination to aid in targeted mineral exploration in the metamorphosed and glaciated terrain that hosts the Rajapalot gold–cobalt prospect. The authors utilise in situ laser ablation inductively coupled plasma mass spectrometry (LA-ICP-MS) techniques for whole rock geochemistry combined with trace element and sulphur isotope compositions of pyrites in the prospect, followed by multivariate statistical data analysis. The high-resolution measurements allowed discrimination to be made between mineralization stages and the cobalt-only and cobalt-gold zones, highlighting the potential of these geochemical applications for the identification of future targets. The LA-ICP-MS approach is recognised as a faster approach than conventional whole rock geochemical determination methods, with the potential for such techniques to be incorporated as standard analytical techniques in the early stages of mineral exploration.
The final contribution to this special issue is the work by Gisbert et al. (2021), who explore the mineral zonation and whole rock geochemical characterization of the volcanic-rock-hosted replacive volcanogenic massive sulfide Aguas Teñidas deposit of the northern Iberian Pyrite Belt (Spain), by means of portable X-ray fluorescence (p-XRF) analysis. The spatial distribution of minerals, as well as major and trace element contents, shows distinct concentration trends in relation to the mineralization zones, with vectors and designed adaptations to the p-XRF approaches used to rapidly analyse unprepared, rough surface hand specimens, contributing to an improved understanding of vectors to ore in replacive-type VMS deposits. The identification of specific lithological units through this technique represents a powerful exploration tool in heavily tectonised areas such as the Iberian Pyrite Belt, as well as contributing key information related to the genesis and evolution of these deposit types globally.
One of the most significant contributions of Earth scientists to the major climate challenges of the 21st century is to facilitate responsible access to minerals. Sustainable exploitation of minerals is needed to support the urgent transition to a low carbon economy (Valero et al., 2018) and meet the increasing need of high-tech metals for advanced computing and engineering (Wellmer et al., 2019; Cheng et al., 2021). The economics of mineral resources have some special features, such as that the resources are where they are – and thus cannot be delocalised – and that the conversion of an exploration target into a fully operating mine can take decades. Thus, mining is an economic activity that is much less flexible than many other sectors.
The major problem is not only to supply the so-called “critical metals” to the society, but to also be able to continue producing the vast amounts of base metals that our society needs. In their current state of implementation, recycling and replacement are not independent alternatives in the near future to meet the high societal demand for minerals, and, although mineral resources are becoming increasingly difficult to source and extract, people rightly continue to demand technologies that improve society, necessitating improvements and innovations within mineral exploration. Many sectors of society demand the materials they need, but also demand that exploration and exploitation of natural resources be done with the best environmental standards, while other sectors prioritise the least social impact to keep costs as low as possible.
New exploration methods are a critical component of smart mining. Less invasive exploration using mineral system models, pathfinders and vectors to ore and advanced geophysics will allow to define more accurately the most promising deep targets saving significant costs in drilling and reducing the social and environmental stress.
It is increasingly difficult to find cropping out or shallow mineral deposits and exploration is evolving towards more and more sophisticated predictive models for finding deposits in the uppermost km of the continental and marine crust. Combined mineral system models, geology, and geophysics try to predict where deposits with the highest resources occur. Barren drillholes and outcrops can provide pathfinders and vectors to ore, also predicting the geological volumes where deposits have more chances to occur. All this is accompanied by new methods of core logging and sophisticated computing including 3D modelling and machine learning. Furthermore, such methods can also be utilised to target active and historic tailings dams at mine sites, where millions of tonnes of “waste” material have accumulated over years (on a scale of decades up to centuries; Bullock et al., 2022) following the separation of the economic rock fraction from the uneconomic minerals. Thanks to improvements in the separation and recovery of economic fractions during the concentration process employed at mine sites, and increasing demand for base metals, precious metals, critical metals and other wanted commodities, historic materials that were once deemed uneconomic may now host targetable resources, without the need to develop new exploration areas or energy-intensive excavation and blasting activities. Determining the resource potential, economic feasibility and specific localities of potential targets within mine waste streams will require cost-effective, non-invasive methods, such as those outlined in this issue.
Is very likely that upcoming decades will see a flourishment of the Earth sciences applied to mineral exploration. Finding new deposits is becoming a challenge not only for the classical exploration geologists – who will probably continue to do field work – but also for other geoscientists and data processing specialists. Mineral prospectors today must search in larger areas, deeper underground, and at higher resolution to find and reach the elusive ore. To meet these challenges, multidisciplinary work is essential.
Publisher’s note: Copernicus Publications remains neutral with regard to jurisdictional claims in published maps and institutional affiliations.
We are grateful to all the contributing authors for submitting their work to the “State of the art in mineral exploration” special issue and to all reviewers for their feedback on the manuscripts. We are also grateful to the Solid Earth executive editors CharLotte Krawczyk and Susanne Buiter for their feedback on this manuscript and the Copernicus editorial office staff for their guidance and help in making the special issue possible.
Juan Alcalde is funded by the Spanish Ministry of Science and Innovation through the Juan de la Cierva fellowship (IJC 2018-036074-I), funded by MCIN/AEI /10.13039/501100011033. Juan Alcalde, Ramon Carbonell and Alba Gil were funded under the EIT-RawMaterials project 17024 (SIT4ME: Seismic Imaging Techniques for Mineral Exploration). Fernando Tornos and Solveig Pospiech are funded by the NEXT (New Exploration Technologies, Contract 776804 H2020). Liam A. Bullock is funded under H2020-EU.1.3.2. (DETAILS Project, grant agreement ID: 101018312).
Adiri, Z., Lhissou, R., El Harti, A., Jellouli, A., and Chakouri, M.: Recent advances in the use of public domain satellite imagery for mineral exploration: A review of Landsat-8 and Sentinel-2 applications, Ore Geol. Rev., 117, 103332, https://doi.org/10.1016/j.oregeorev.2020.103332, 2020.
Alcalde, J., Martí, D., Juhlin, C., Malehmir, A., Sopher, D., Saura, E., Marzán, I., Ayarza, P., Calahorrano, A., Pérez-Estaún, A., and Carbonell, R.: 3-D reflection seismic imaging of the Hontomín structure in the Basque–Cantabrian Basin (Spain), Solid Earth, 4, 481–496, https://doi.org/10.5194/se-4-481-2013, 2013.
Alcalde, J., Carbonell, R., Malehmir, A., Gil, A., Buske, S., Orlowsky, D., Hupe, T., Ayarza, P., Martínez, Y., and Tornos, F.: SIT4ME – Seismic Imaging for Mineral Exploration, NSG2020 3rd Conference on Geophysics for Mineral Exploration and Mining, European Association of Geoscientists & Engineers, 1–5, 7–8 December 2020, online, https://doi.org/10.3997/2214-4609.202020072, 2020.
Alcalde, J., Heinemann, N., James, A., Bond, C. E., Ghanbari, S., Mackay, E. J., Haszeldine, R. S., Faulkner, D. R., Worden, R. H., and Allen, M. J. A: criteria-driven approach to the CO2 storage site selection of East Mey for the acorn project in the North Sea, Mar. Petrol. Geol., 133, 105309, https://doi.org/10.1016/j.marpetgeo.2021.105309, 2021.
Ali, S. H., Giurco, D., Arndt, N., Nickless, E., Brown, G., Demetriades, A., Durrheim, R., Enriquez, M. A., Kinnaird, J., Littleboy, A., Meinert, L. D., Oberhänsli, R., Salem, J., Schodde, R., Schneider, G., Vidal, O., and Yakovleva, N.: Mineral supply for sustainable development requires resource governance, Nature, 543, 367–372, https://doi.org/10.1038/nature21359, 2017.
Aranha, M., Porwal, A., Sundaralingam, M., González-Álvarez, I., Markan, A., and Rao, K.: Rare earth elements associated with carbonatite–alkaline complexes in western Rajasthan, India: exploration targeting at regional scale, Solid Earth, 13, 497–518, https://doi.org/10.5194/se-13-497-2022, 2022.
Bellefleur, G., Malinowski, M., and Urosevic, M.: Editorial for Special Issue “Seismic Methods in Mineral Exploration”, Minerals, 9, 630, https://doi.org/10.3390/min9100630, 2019.
Boullenger, B., Verdel, A., Paap, B., Thorbecke, J., and Draganov, D.: Studying CO2 storage with ambient-noise seismic interferometry: A combined numerical feasibility study and field-data example for Ketzin, Germany, Geophysics, 80, Q1–Q13, https://doi.org/10.1190/geo2014-0181.1, 2015.
Bullock, L. A., Yang, A., and Darton, R. C.: Kinetics-informed global assessment of mine tailings for CO2 removal, Sci. Total Environ., 808, 152111, https://doi.org/10.1016/j.scitotenv.2021.152111, 2022.
Chamarczuk, M., Malinowski, M., Draganov, D., Koivisto, E., Heinonen, S., and Rötsä, S.: Reflection imaging of complex geology in a crystalline environment using virtual-source seismology: case study from the Kylylahti polymetallic mine, Finland, Solid Earth, 13, 705–723, https://doi.org/10.5194/se-13-705-2022, 2022.
Cheng, X., Liu, H., Yuan, H., Peng, H., Tang, C., Huang, J., and Zhang, Q. A.: perspective on sustainable energy materials for lithium batteries, SusMat, 1, 38–50, https://doi.org/10.1002/sus2.4, 2021.
Cheraghi, S., Craven, J. A., and Bellefleur, G.: Feasibility of virtual source reflection seismology using interferometry for mineral exploration: A test study in the Lalor Lake volcanogenic massive sulphide mining area, Manitoba, Canada, Geophys. Prospect., 63, 833–848, https://doi.org/10.1111/1365-2478.12244, 2015.
Cheraghi, S., Malehmir, A., Naghizadeh, M., Snyder, D., Mathieu, L., and Bedeaux, P.: Seismic imaging across fault systems in the Abitibi greenstone belt – an analysis of pre- and post-stack migration approaches in the Chibougamau area, Quebec, Canada, Solid Earth, 12, 1143–1164, https://doi.org/10.5194/se-12-1143-2021, 2021.
Colombero, C., Papadopoulou, M., Kauti, T., Skyttä, P., Koivisto, E., Savolainen, M., and Socco, L. V.: Surface-wave tomography for mineral exploration: a successful combination of passive and active data (Siilinjärvi phosphorus mine, Finland), Solid Earth, 13, 417–429, https://doi.org/10.5194/se-13-417-2022, 2022.
Da Col, F., Papadopoulou, M., Koivisto, E., Sito, Ł., Savolainen, M., and Socco, L. V.: Application of surface-wave tomography to mineral exploration: a case study from Siilinjärvi, Finland, Geophys. Prospect., 68, 254–269, https://doi.org/10.1111/1365-2478.12903, 2020.
Dentith, M., Yuan, H., Johnson, S., Murdie, R., and Piña-Varas, P.: Application of deep-penetrating geophysical methods to mineral exploration: Examples from Western Australia, Geophysics, 83, WC29–WC41, https://doi.org/10.1190/geo2017-0482.1, 2018.
Ding, Y. and Malehmir, A.: Reverse time migration (RTM) imaging of iron oxide deposits in the Ludvika mining area, Sweden, Solid Earth, 12, 1707–1718, https://doi.org/10.5194/se-12-1707-2021, 2021.
Eaton, D. W., Milkereit, B., and Salisbury, M.: Seismic methods for deep mineral exploration: Mature technologies adapted to new targets, The Leading Edge, 22, 580–585, https://doi.org/10.1190/1.1587683, 2003.
Gil, A., Malehmir, A., Buske, S., Alcalde, J., Ayarza, P., Martínez, Y., Lindskog, L., Spicer, B., Carbonell, R., Orlowsky, D., Carriedo, J., and Hagerud, A.: Reflection seismic imaging to unravel subsurface geological structures of the Zinkgruvan mining area, central Sweden, Ore Geol. Rev., 137, 104306, https://doi.org/10.1016/j.oregeorev.2021.104306, 2021.
Gisbert, G., Tornos, F., Losantos, E., Pons, J. M., and Videira, J. C.: Vectors to ore in replacive volcanogenic massive sulfide (VMS) deposits of the northern Iberian Pyrite Belt: mineral zoning, whole rock geochemistry, and application of portable X-ray fluorescence, Solid Earth, 12, 1931–1966, https://doi.org/10.5194/se-12-1931-2021, 2021.
Hloušek, F., Malinowski, M., Bräunig, L., Buske, S., Malehmir, A., Markovic, M., Sito, L., Marsden, P., and Bäckström, E.: Three-dimensional reflection seismic imaging of the iron oxide deposits in the Ludvika mining area, Sweden, using Fresnel volume migration, Solid Earth, 13, 917–934, https://doi.org/10.5194/se-13-917-2022, 2022.
Ikelle, L. T. and Amundsen, L.: Introduction to Petroleum Seismology, Introduction to Petroleum Seismology, Society of Exploration Geophysicists, Investigations in Geophysics Series No. 12, 1374 pp., https://doi.org/10.1190/1.9781560803447, 2018.
Jackisch, R., Heincke, B. H., Zimmermann, R., Sørensen, E. V., Pirttijärvi, M., Kirsch, M., Salmirinne, H., Lode, S., Kuronen, U., and Gloaguen, R.: Drone-based magnetic and multispectral surveys to develop a 3D model for mineral exploration at Qullissat, Disko Island, Greenland, Solid Earth, 13, 793–825, https://doi.org/10.5194/se-13-793-2022, 2022.
Kelka, U., Martinez, C., Krapf, C., Westerlund, S., Gonzalez-Alvarez, I., Pawley, M., and Foss, C.: Establishing an integrated workflow identifying and linking surface and subsurface lineaments for mineral exploration under cover: example from the Gawler Craton, South Australia, Solid Earth, 13, 827–847, https://doi.org/10.5194/se-13-827-2022, 2022.
Kirsch, M., Lorenz, S., Zimmermann, R., Tusa, L., Möckel, R., Hödl, P., Booysen, R., Khodadadzadeh, M., and Gloaguen, R.: Integration of Terrestrial and Drone-Borne Hyperspectral and Photogrammetric Sensing Methods for Exploration Mapping and Mining Monitoring, Remote Sens., 10, 1366, https://doi.org/10.3390/rs10091366, 2018.
Lopes, L., Bodo, B., Rossi, C., Henley, S., Žibret, G., Kot-Niewiadomska, A., and Correia, V.: ROBOMINERS – Developing a bio-inspired modular robot-miner for difficult to access mineral deposits, Adv. Geosci., 54, 99–108, https://doi.org/10.5194/adgeo-54-99-2020, 2020.
Malehmir, A., Durrheim, R., Bellefleur, G., Urosevic, M., Juhlin, C., White, D. J., Milkereit, B., and Campbell, G.: Seismic methods in mineral exploration and mine planning: A general overview of past and present case histories and a look into the future, Geophysics, 77, WC173–WC190, https://doi.org/10.1190/geo2012-0028.1, 2012.
Malehmir, A., Manzi, M., Draganov, D., Weckmann, U., and Auken, E.: Introduction to the special issue on “Cost-effective and innovative mineral exploration solutions”, Geophys. Prospect, 68, 3–6, https://doi.org/10.1111/1365-2478.12915, 2020.
Malehmir, A., Markovic, M., Marsden, P., Gil, A., Buske, S., Sito, L., Bäckström, E., Sadeghi, M., and Luth, S.: Sparse 3D reflection seismic survey for deep-targeting iron oxide deposits and their host rocks, Ludvika Mines, Sweden, Solid Earth, 12, 483–502, https://doi.org/10.5194/se-12-483-2021, 2021.
Marjoribanks, R.: Geological Methods in Mineral Exploration and Mining, Springer, Berlin, Heidelberg, 238 pp., https://doi.org/10.1007/978-3-540-74375-0, 2010.
Markovic, M., Maries, G., Malehmir, A., Ketelhodt, J., Bäckström, E., Schön, M., and Marsden, P.: Deep reflection seismic imaging of iron-oxide deposits in the Ludvika mining area of central Sweden, Geophys. Prospect., 68, 7–23, https://doi.org/10.1111/1365-2478.12855, 2020.
Martinez, Y., Alcalde, J., Marti, D., Ayarza, P., Ruiz, M., Marzán, I., Tornos, F., Malehmir, A., Gil, A., Buske, S., Orlowsky, D., and Carbonell, R.: Reflection Seismic Imaging for Mineral Exploration in the Sotiel-Coronada Area, Southwest Spain, NSG2020 3rd Conference on Geophysics for Mineral Exploration and Mining, European Association of Geoscientists & Engineers, 1–5, 7–8 December 2020, online, https://doi.org/10.3997/2214-4609.202020148, 2020.
Meinert, L. D., Robinson, G. R., and Nassar, N. T.: Mineral resources: Reserves, peak production and the future, Resources, 5, 14 pp., https://doi.org/10.3390/resources5010014, 2016.
Naghizadeh, M., Snyder, D., Cheraghi, S., Foster, S., Cilensek, S., Floreani, E., and Mackie, J.: Acquisition and Processing of Wider Bandwidth Seismic Data in Crystalline Crust: Progress with the Metal Earth Project, Minerals 9, 145, https://doi.org/10.3390/min9030145, 2019.
Raič, S., Molnár, F., Cook, N., O'Brien, H., and Lahaye, Y.: Application of lithogeochemical and pyrite trace element data for the determination of vectors to ore in the Raja Au–Co prospect, northern Finland, Solid Earth, 13, 271–299, https://doi.org/10.5194/se-13-271-2022, 2022.
Romero, P. and Schimmel, M.: Mapping the Basement of the Ebro Basin in Spain With Seismic Ambient Noise Autocorrelations, J. Geophys. Res.-Sol. Ea., 123, 5052–5067, https://doi.org/10.1029/2018JB015498, 2018.
Ryberg, T., Kirsch, M., Haberland, C., Tolosana-Delgado, R., Viezzoli, A., and Gloaguen, R.: Ambient seismic noise analysis of LARGE-N data for mineral exploration in the Central Erzgebirge, Germany, Solid Earth, 13, 519–533, https://doi.org/10.5194/se-13-519-2022, 2022.
Sánchez-Pastor, P., Obermann, A., Schimmel, M., Weemstra, C., Verdel, A., and Jousset, P.: Short- and Long-Term Variations in the Reykjanes Geothermal Reservoir From Seismic Noise Interferometry, Geophys. Res. Lett., 46, 5788–5798, https://doi.org/10.1029/2019GL082352, 2019.
Schodde, R. C.: Key issues affecting the time delay between discovery and development – is it getting harder and longer?, presented to Prospectors and Developers Association of Canada (PDAC) Conference, 3 March, Toronto, Canada, 2014.
Sena-Lozoya, E. B., González-Escobar, M., Gómez-Arias, E., González-Fernández, A., and Gómez-Ávila, M.: Seismic exploration survey northeast of the Tres Virgenes Geothermal Field, Baja California Sur, Mexico: A new Geothermal prospect, Geothermics, 84, 101743, https://doi.org/10.1016/j.geothermics.2019.101743, 2020.
Singh, B., Malinowski, M., Górszczyk, A., Malehmir, A., Buske, S., Sito, Ł., and Marsden, P.: 3D high-resolution seismic imaging of the iron oxide deposits in Ludvika (Sweden) using full-waveform inversion and reverse time migration, Solid Earth, 13, 1065–1085, https://doi.org/10.5194/se-13-1065-2022, 2022.
Valero, A., Valero, A., Calvo, G., Ortego, A., Ascaso, S., and Palacios, J.-L.: Global material requirements for the energy transition. An exergy flow analysis of decarbonisation pathways, Energy, 159, 1175–1184, https://doi.org/10.1016/j.energy.2018.06.149, 2018.
Villalobos-García, R. and Odriozola-Lloret, C. P.: Prehistoric mining tools of the variscite mines of Palazuelo de las Cuevas (Zamora) and Pico Centeno (Huelva). A comparative analysis, Zephyrvs, 77, 79, https://doi.org/10.14201/zephyrus2016777998, 2016.
Wellmer, F.-W., Buchholz, P., Gutzmer, J., Hagelüken, C., Herzig, P., Littke, R., and Thauer, R. K.: Supply of Raw Materials and Effects of the Global Economy. Raw Materials for Future Energy Supply, Springer International Publishing, Cham, 23–105, https://doi.org/10.1007/978-3-319-91229-5_3, 2019.
Xie, T., Xu, T., Ai, Y., Zeng, Q., Zhang, W., and Zheng, F.: Imaging the shallow crustal velocity structure of the Qingchengzi ore field on the Liaodong Peninsula, China, with a short-period dense array using ambient noise tomography, Tectonophysics 813, 228913, https://doi.org/10.1016/j.tecto.2021.228913, 2021.
Yousefi, M., Kreuzer, O. P., Nykänen, V., and Hronsky, J. M. A.: Exploration information systems – A proposal for the future use of GIS in mineral exploration targeting, Ore Geol. Rev., 111, 103005, https://doi.org/10.1016/j.oregeorev.2019.103005, 2019.
Zuo, R. and Xiong, Y.: Big Data Analytics of Identifying Geochemical Anomalies Supported by Machine Learning Methods, Nat. Resour. Res., 27, 5–13, https://doi.org/10.1007/s11053-017-9357-0, 2018.