the Creative Commons Attribution 4.0 License.
the Creative Commons Attribution 4.0 License.
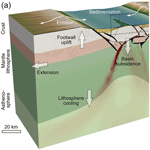
(D)rifting in the 21st century: key processes, natural hazards, and geo-resources
Patricia Cadenas
Mohamed Gouiza
Jordan J. J. Phethean
Sascha Brune
Anne C. Glerum
Rifting and continental break-up are major research topics within geosciences, and a thorough understanding of the processes involved as well as of the associated natural hazards and natural resources is of great importance to both science and society. As a result, a large body of knowledge is available in the literature, with most of this previous research being focused on tectonic and geodynamic processes and their links to the evolution of rift systems. We believe that the key task for researchers is to make our knowledge of rift systems available and applicable to face current and future societal challenges. In particular, we should embrace a system analysis approach and aim to apply our knowledge to better understand the links between rift processes, natural hazards, and the geo-resources that are of critical importance to realise the energy transition and a sustainable future. The aim of this paper is therefore to provide a first-order framework for such an approach by providing an up-to-date summary of rifting processes, hazards, and geo-resources, followed by an assessment of future challenges and opportunities for research. We address the varied terminology used to characterise rifting in the scientific literature, followed by a description of rifting processes with a focus on the impact of (1) rheology and stain rates, (2) inheritance in three dimensions, (3) magmatism, and (4) surface processes. Subsequently, we describe the considerable natural hazards that occur in rift settings, which are linked to (1) seismicity, (2) magmatism, and (3) mass wasting, and provide some insights into how the impacts of these hazards can be mitigated. Moreover, we classify and describe the geo-resources occurring in rift environments as (1) non-energy resources, (2) geo-energy resources, (3) water and soils, and (4) opportunities for geological storage. Finally, we discuss the main challenges for the future linked to the aforementioned themes and identify numerous opportunities for follow-up research and knowledge application. In particular, we see great potential in systematic knowledge transfer and collaboration between researchers, industry partners, and government bodies, which may be the key to future successes and advancements.
- Article
(12168 KB) - Full-text XML
- BibTeX
- EndNote
The surface of our planet is in perpetual motion as the Earth's continents continuously converge and diverge, assembling supercontinents through subduction and collision or separating them through rifting and continental break-up processes. This constant assembly and dismantling of continents, known as the Wilson Cycle (e.g. Wilson, 1966, 1968; Wilson et al., 2019) (Fig. 1), is driven by plate tectonic forces that act on the Earth's lithosphere (i.e. the rigid outermost layer of our planet made up of tectonic plates, situated above the asthenospheric mantle). Subduction and collision of tectonic plates during the convergence phase of the Wilson Cycle have created the world's mountain ranges and deep oceanic trenches. By contrast, rifting and continental break-up during the divergence phase of the Wilson Cycle involve the initial formation of individual rift basins, which can gradually link up to evolve into large-scale rift systems. They may ultimately form oceanic basins when break-up of the continental lithosphere takes place. These oceanic basins are flanked by rifted continental margins on either side and comprise a mid-oceanic spreading ridge in between them, along which new oceanic lithosphere is generated (Fig. 1).
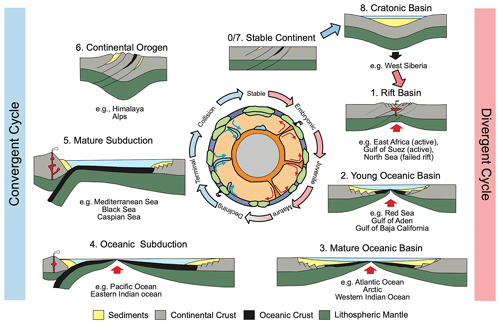
Figure 1Schematic representation of the Wilson Cycle, as originally proposed by Wilson (1968). Note that the asthenosphere below the lithospheric mantle is not depicted. Image modified after Wilson et al. (2019).
A detailed understanding of the geodynamic processes involved in rifting and continental break-up is of great scientific, economic, and societal relevance. Onshore and offshore rift basins contain extensive sedimentary accumulations that archive crucial information regarding past local and global environmental changes and vast amounts of geo-resources, i.e. natural resources such as hydrocarbons, geothermal energy, helium and natural hydrogen gas (H2), mineral and non-mineral deposits, fresh (ground)water, and fertile soils (e.g. Catuneanu et al., 2009; Davison and Underhill, 2013; Zappettini et al., 2017). On the other hand, rift basins cover large swaths of the Earth's surface (Fig. 2) and present significant hazards in relation to volcanism, earthquakes, and (submarine) landslides, especially since large populations are concentrated in such environments (e.g. Brune, 2016). In fact, natural disasters in rift environments have caused extensive damage and claimed many lives over the course of human history (e.g. Gouin, 1979; Liu et al., 2007; Hearn, 2022).
Due to the importance of rifting, an extensive body of scientific literature is available on the topic, summarised and reviewed by numerous authors (e.g. Bradley, 2008; Corti, 2012; Roberts and Bally, 2012; Franke, 2013; Nemčok, 2016; Alves et al., 2020; Şengör, 2020; Buiter et al., 2023; Brune et al., 2023; Peron-Pinvidic, 2022; Pérez-Gussinyé et al., 2023). Most of these authors have focused on tectonic and geodynamic processes and their links to the evolution of rift systems. However, we believe that the most important challenge that lies ahead for researchers is to make our knowledge of rift systems available and applicable to new societal challenges. In particular, we should aim to apply our knowledge to better understand the links between rift processes, natural hazards, and the geo-resources that are of critical importance to the global transition towards a sustainable future economy.
The aim of this paper is therefore to provide an up-to-date overview of our knowledge of rifting processes with this challenge in mind. We first describe the various definitions used to analyse the evolution of rift systems from inception to break-up and drifting. Subsequently, we focus on the key processes that control the evolution of rift systems and link these processes to the occurrence and development of natural hazards and geo-resources in such systems. By doing so, we explore key challenges and opportunities for new research efforts and knowledge application, and we hope that this paper as a whole will serve as a guide, inspiring upcoming projects in the field of rift research.
In this section we summarise the key processes causing and influencing the development of rift systems. We start by defining the terminology applied in the field before addressing how the lithosphere is deformed as a result of rifting. We subsequently explore the impact of structural inheritance and the need to understand rift systems in a 4D framework (i.e. in time and space). The final topics we treat are the impacts of magmatism and surface processes on the evolution of rift systems.
2.1 Terminology
The study of rifting has a long history, reaching back to the late 1500s, when cartographers first noticed the apparent fit between the coastlines on both sides of the South Atlantic (Romm, 1994). Since then, our understanding of the natural world has greatly advanced, and a broad variety of methods has been applied to study rifting over the past centuries. These methods include geological mapping and sampling, borehole logging, interpretation of 2D and 3D seismic and other regional geophysical datasets, and aerial and satellite observation, as well as analogue and numerical modelling of rifting processes. As a consequence of using different analytical and exploratory methods in distinct areas of the world, researchers have historically developed a plethora of overlapping terminology to describe rifting processes and the different stages of rift evolution, which range from initial thinning of the lithosphere and the associated formation of rift basins to the eventual development of rifted margins flanking oceanic basins (e.g. Corti, 2012; Péron-Pinvidic and Manatschal, 2009) (Fig. 3).
In this paper, the term “rifting” refers to the development of an extensional tectonic setting due to divergent tectonic plate motion, be it in a continental or oceanic environment (i.e. before and after break-up of the continental lithosphere and the establishment of an oceanic lithosphere, respectively). Similarly, we use the term “rift system”, “rift environment”, or “rift settings” for extensional tectonic systems in both continental and oceanic contexts. We also apply the broad term “rifted margin”, where synonyms are “passive margins” (in contrast to active subduction margins, even though “passive” rifted margins are often actively deforming), “extensional margins”, “divergent margins”, or “Atlantic margins” (in contrast to the Pacific subduction margins). Moreover, we essentially adopt the general classification used by Péron-Pinvidic and Manatschal (2009), recognising five main stages during the evolution of rift systems (Fig. 3). These five main stages serve as a robust first-order framework throughout this work:
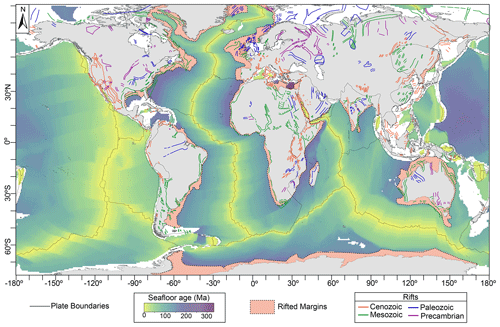
Figure 2Global distribution of continental rift basins and rifted margins. Rift distribution after Şengör (2020). Rifted continental margins after Berndt et al. (2019). Plate boundaries from Bird (2003) taken from https://www.earthbyte.org (last access: 11 July 2024). Seafloor age from Müller et al. (2019) and Seton et al. (2020).
-
The initial state of the system prior to rift initiation is defined as Stage 0 (pre-rift).
-
As soon as rifting is initiated, the system moves into Stage 1 (stretching), when extension is accommodated by distributed deformation of the continental lithosphere, leading to widespread normal faulting at the surface.
-
During the subsequent Stage 2 (necking), extension begins to concentrate, causing localised thinning of the continental lithosphere, the development of distinct rift basins, and a marked rise in the mantle below.
-
The start of Stage 3 (break-up) is marked by the separation of crustal layers following hyperextension (high degrees of crustal thinning) of the rifted margins, exhumation of mantle material to (or near to) the surface, and the development of the continent–ocean transition (COT) when the first oceanic lithosphere is formed.
-
Finally, Stage 4 (drifting) represents rifting after the establishment of a mid-oceanic spreading ridge and the development of an oceanic lithosphere. Rifted continental margins are fully developed by this stage and generally record regional-scale subsidence and seaward tilting due to both cooling of the lithosphere and loading induced by the development of extensive sediment deposits.
It must be noted that we regard these five stages as being representative of the general evolution of rift systems, independent of the tectonic environment in which they develop; rifting may be initiated by either asthenospheric upwelling (active rifting) due to, for example, mantle plume activity or slab break-off, or by far-field stresses (passive rifting) due to subduction rollback or continental collision. These different causes initiating rifting can overlap in space and time and have inspired multiple rift classifications in the literature (e.g. Merle, 2011; Şengör, 2020; Peron-Pinvidic, 2022), yet the expression of rifting processes generally follows the same general trend as outlined above.
Even so, lithospheric extension may halt at any stage prior to the drifting phase, resulting in so-called failed rifts (aulacogens), which nonetheless are affected by post-rift thermal sagging. If a subsequent phase of convergence ensues, tectonic reactivation and basin inversion occurs in such failed rifts (e.g. Zwaan et al., 2022, and references therein), and, if this convergence continues in time, a short-cut version of the Wilson Cycle may be established (Chenin et al., 2019). Furthermore, various “passive” rifted margins are unusually uplifted due to mantle processes (e.g. South African and Afar–Red Sea margins; Lithgow-Bertelloni and Silver, 1998; Hassan et al., 2020), magmatic underplating (e.g. the Brazilian, Madagascar, and western Indian margins; Gunnell and Fleitout, 1998; Mohriak et al., 2008; Radhakrishna et al., 2019), plate convergence (e.g. the Moroccan Atlas and its inverted rift basins; Benabdellouahed et al., 2017), or glacial rebound after the recent removal of thick ice sheets (e.g. the Norwegian margin; Bungum et al., 2010).
In the literature, another key distinction has been established between magma-rich and magma-poor rift systems, since large-scale magmatism, often linked to mantle plume activity, has a significant impact on rifting per se and on the architecture of rifted margins (e.g. Franke, 2013; Buck, 2017; Sapin et al., 2021). This distinction is further explored in Sect. 2.4.
2.2 Impact of rheology and strain rate
The type of lithospheric deformation occurring in a rift system will largely control its evolution. The general type of deformation is broadly dependent on the interplay between lithospheric rheology, timing (the specific rifting stage), and plate motion velocities. Within this context, rifting is characterised by thinning of the lithosphere, accommodated by either ductile flow or brittle normal faulting.
Firstly, the overall rheology of the lithosphere is strongly impacted by the presence of weak ductile layers in the lithosphere, most significant of which is the ductile lower crust (e.g. Brun, 1999; Burov and Watts, 2006; Fig. 4), though clay and evaporite layers can have a similar effect on a smaller scale (Sect. 2.5). When present, as in standard continental lithosphere with some 40 km of crust on top of 100 km mantle lithosphere, such a layer can decouple brittle deformation in the mantle lithosphere, which represents the strongest (i.e. most competent) part of the lithosphere, from brittle deformation in the overlying, competent upper crust. Increased decoupling in a hotter lithosphere means that deformation is free to localise throughout the upper crust and parts of the upper lithospheric mantle, leading to distributed or wide rifting and even core complex development (e.g. in the Basin and Range Province in the USA or in the Aegean Sea; Kydonakis et al., 2015; Brun et al., 2018; Fig. 4). By contrast, in systems with a thin or non-existent weak ductile lower crust, such as cold cratonic lithosphere, most (if not all) of the lithospheric column is strong and brittle, rendering structural decoupling a minor factor. As a result, the deformation type is fully dictated by the mantle lithosphere and tends to be localised (narrow rifting, e.g. Buck, 1991; Brun, 1999; Brune, 2016) or simply occurs wherever the lithosphere is locally weakened, as documented in the East African Rift System (see Sect. 2.3).
Secondly, the progression of rifting is of importance as the rheology of the lithosphere changes over time. A very thick ductile lower crust that can lead to core complex formation is characteristic of systems with a thickened crust, for instance, after the development of a mountain range in which a surplus of radiogenic heating occurs prior to rifting, i.e. during the pre-rift stage (Figs. 3 and 4) (Buck, 1991; Brun et al., 2018). A typical continental lithosphere containing a weak ductile lower crust promotes a well-distributed deformation style, characteristic of the first rifting stage (stretching stage; Fig. 3). As rifting progresses, the lithospheric layers, including the lower crust, start to thin, so the decoupling effect decreases in importance and the mantle influence on upper crustal deformation starts to increase, leading to a strongly localised stretching regime, heralding the start of the second rifting stage (necking stage; Fig. 3). During this stage, we also tend to observe a shift from initial symmetric, pure shear rift geometries to asymmetric, simple shear rift geometry (Lavier and Manatschal, 2006) (Fig. 4), although magmatism can prevent this shift from occurring. With continued rifting, the crustal layers will be broken apart to give way to the rising mantle so that the influence of the weak ductile lower crust will be negligible from this point in time (break-up stage; Fig. 3). Deformation in an oceanic rift system (drifting stage; Fig. 3) is then mostly controlled by the degree to which extension is accommodated by magmatic intrusions (Buck et al., 2005).
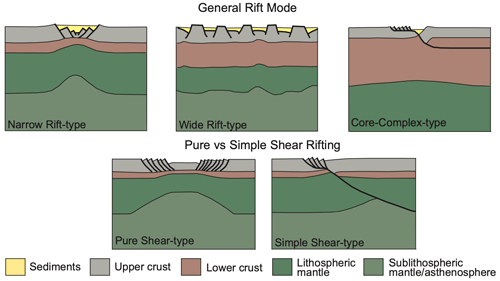
The influence of strain rate is expressed in the altered behaviour of ductile layers in the lithosphere compared to its brittle parts above given that the brittle deformation of competent lithospheric layers is not dependent on the strain rate. When strain rates are low, ductile materials in the lithosphere generally weaken, whereas higher strain rates cause them to become more competent (e.g. Brun, 1999). This has a direct impact on the decoupling caused by a weak ductile lower crust during the stretching and necking stages of a rift system: slower plate motion tends to reduce coupling between brittle and ductile parts of the lithosphere, promoting wide rifting, whereas faster plate motion increases coupling between brittle and ductile lithospheric rock, promoting narrow rifting (Fig. 4). However, feedbacks between thermal and mechanical processes render the process more complex: at a low extension rate, when the rift centre has sufficient time to cool either through conduction or hydrothermal circulation, rift strength may increase and eventually lead to relocalisation of the rift centre or to the abandonment of the rift branch (van Wijk and Cloetingh, 2002). During the drifting stage, ultraslow plate divergence (v<10 mm yr−1; Phethean et al., 2022) can allow oceanic core complex formation (Brun et al., 2018), for example, along the SW Indian Ridge and the Gakkel Ridge in the Arctic Ocean (Dick et al., 2003; Zhou et al., 2022). Slow-spreading (10–40 mm yr−1) ridges can also host core complexes but tend to develop more typical mid-oceanic ridges with well-defined axial valleys, as observed along the Mid-Atlantic Ridge (Macdonald, 2019). By contrast, faster plate motion (>90 mm yr−1) during the drifting stage generally leads to axial highs that are elevated several hundreds of metres, for instance, along the mid-oceanic ridges of the Pacific (Macdonald, 2019). However, this general relation between plate divergence rate and mid-oceanic ridge morphology type does not always hold, since the morphology of mid-oceanic ridges can be significantly altered depending on rates of magma supply (Cannat et al., 2006; Macdonald, 2019). It should also be noted that plate motion rates in various rifts (e.g. the Atlantic rift, the Australia–Antarctica rift, and the South China Sea) tend to strongly increase some 10–20 Myr prior to continental break-up (Brune, 2016). This increase in plate motion velocity from ca. 5 mm yr−1 (e.g. in the present-day continental East African Rift System; Stamps et al., 2021) up to some 20 mm yr−1 or more (e.g. in the Red Sea and Gulf of Aden oceanic basins; ArRajehi et al., 2010) is linked to strong weakening of the overall lithosphere during the preceding necking stage. Consequently, any resistance to the plate tectonic forces acting on the rift system is largely removed, allowing the plate motion to accelerate so that the plates can attain the “real unrestrained” plate motion velocity typical of rift basins in the drifting stage (Brune et al., 2016).
Furthermore, the spherical shape of the Earth dictates rotational motion of tectonic plates about an Euler pole. Such plate rotation may induce significant gradients in plate divergence velocities along plate boundaries and thus along rift systems (e.g. ArRajehi et al., 2010; Stamps et al., 2021), with various impacts on the development of rift segments. Firstly, these plate divergence gradients induce rift basin formation far away from the Euler pole, followed by rift basin propagation towards this same pole, as observed along the East African Rift System (e.g. Biggs et al., 2021; Zwaan and Schreurs, 2023). Secondly, such plate divergence gradients cause along-strike differences in tectonic style. For instance, the aforementioned ultraslow Gakkel Ridge with its core complexes is close to the North America–Eurasia Euler pole, whereas further away from the pole divergence velocities increase and we find the well-defined axial valleys of the Mid-Atlantic Ridge (Dick et al., 2003; DeMets et al., 2010; Macdonald, 2019). The Gakkel Ridge also shows an oceanic rift system propagating into continental lithosphere at the Laptev margin, where the relatively well-localised deformation along the ridge is dispersed over various small basins (Franke et al., 2001). Similar settings, where oceanic rifts enter continental lithosphere, are found in the Havre Trough–Taupō Rift system in New Zealand (Benes and Scott, 1996) and in the Woodlark Basin offshore Papua New Guinea (Taylor et al., 1999).
2.3 Inheritance and rifting in 3D
The long and complex history of the Earth's continental lithosphere leaves us with various types of inheritance that weaken the lithosphere and may affect strain localisation during rifting. Structural inheritance can come in the shape of pre-rift structures such as discrete faults or shear zones; pervasive fabrics in basement rocks; variations in lithospheric strength or layering between, for instance, a craton and adjacent terranes; compositional variations due to chemical alteration; or thermal variations due to mantle activity or previous lithospheric thinning (Schiffer et al., 2020; Glerum et al., 2020; Gouiza and Naliboff, 2021; Samsu et al., 2022).
The reactivation of inherited lithospheric structures during rifting depends on various factors. Most importantly, the weakness must sufficiently impact the strength of the lithosphere; pre-existing faults that are poorly developed during an earlier deformation phase are shown to have little impact on subsequent basin development, and vice versa for well-developed faults, as observed in eastern Africa and in the Trans-Mexican Volcanic Belt (e.g. Maestrelli et al., 2020; Wang et al., 2021). Even large-scale suture zones may not always be reactivated, as Wilson (1966) recognised in the North Atlantic realm. In such a setting, the location of weaknesses in the lithosphere and the overall rheology of the lithosphere itself are of importance; when a weak ductile lower crust is present, structural inheritance in the lithospheric mantle and crust may reactivate independently due to structural decoupling (Zwaan et al., 2022). Conversely, in the case of high coupling, inheritance in the competent mantle lithosphere should be expected to have dominant control over the localisation of deformation. Increased structural coupling due to progressive rifting can lead to the overprinting of crustal structures by subsequent mantle-controlled deformation during necking (e.g. Zwaan et al., 2022), an example of which is recorded in the Mesozoic North Sea rift (Erratt et al., 1999).
Moreover, the 3D orientation of the structural inheritance has a large impact; even a well-developed inherited shear zone may not reactivate when not oriented favourably for reactivation in terms of dip and strike with respect to the regional stress field (e.g. Maestrelli et al., 2020; Bonini et al., 2023). As a consequence, the structural inheritance the individual rift segments follow can result in different structural styles along each rift segment, ranging from orthogonal rifts to oblique and even transform systems (e.g. Corti et al., 2007; Agostini et al., 2011). While orthogonal rifts display along-strike faulting, oblique rift systems tend to develop oblique (en echelon or offset) fault systems and rift basins (e.g. the Baikal Rift Zone and the Main Ethiopian Rift; Petit et al., 1996; Agostini et al., 2011). Highly oblique rift systems may develop into transform margins after the break-up stage, as observed along the Knipovich Ridge (Dumais et al., 2020) and the Davis Strait (Hosseinpour et al., 2013), both in the North Atlantic.
Finally, some types of inheritance can actively prevent reactivation. Rifting is often multi-phased, with potentially long periods of tectonic quiescence in between (e.g. Doré et al., 1999). This leaves time for cooling and strengthening of the mantle material that has risen below a rift basin that entered the necking stage, which may prevent the reactivation of that basin when the next rifting phase starts. Instead, the reactivated rift system can jump to localise along a different rift axis, where the lithosphere is weaker, as highlighted by the sequence of pre-break-up rift events with different rift axes in the Mesozoic of the North Atlantic realm (e.g. Doré et al., 1999; Naliboff and Buiter, 2015).
2.4 Magma-rich and magma-poor rifting
Multiple rift systems have experienced extensive magmatism at some point during their development, classifying them as magma-rich systems (in contrast to magma-poor systems; e.g. Franke, 2013; Tugend et al., 2015). Magmatism is often the result of mantle anomalies (Peace et al., 2020), and the timing of magmatism is of key importance to understand its impact on the evolution of rifting, continental break-up, and rifted margin architecture (e.g. Buiter and Torsvik, 2014; Sapin et al., 2021).
Some rift systems experienced magmatism prior to rifting (i.e. during the pre-rift stage). A prime example is found in the Afar Triple Junction area, where extensive flood basalts up to 2 km thick covered large areas of present-day Ethiopia, Eritrea, and Yemen (Mohr, 1983). This outpouring of flood basalts is linked to the arrival of one or multiple mantle plumes below eastern Africa (Hansen et al., 2012; Hassan et al., 2020, and references therein). In general, syn-rift magmatism can take place during all stages of rifting (Peace et al., 2020; Manatschal et al., 2023). A key impact of such syn-rift magmatism is the significant reduction in lithospheric strength that can occur when intruded by magma (Buck, 2006) (Fig. 5). This strength reduction allows the efficient localisation of deformation along the rift axis, accompanied by only limited faulting, altogether referred to as “magma-accommodated rifting”, which allows rifting to remain rather symmetrical. Deformation along large parts of the rift axes in the Ethiopian Rift and Afar region is considered magma-accommodated and has a very specific style, which can also be found in Iceland (Rime et al., 2023, and references therein). The Afar region also shows the various stages of magma-rich rifting up to ongoing break-up along the so-called magmatic segments that mark the rift axes (Varet, 2018). On the other hand, (mafic) intrusions, when allowed to cool and solidify, can strengthen the lithosphere (Liu and Furlong, 1994), which is somewhat similar to the cooling of shallow mantle material below failed rifts (see Sect. 2.3).
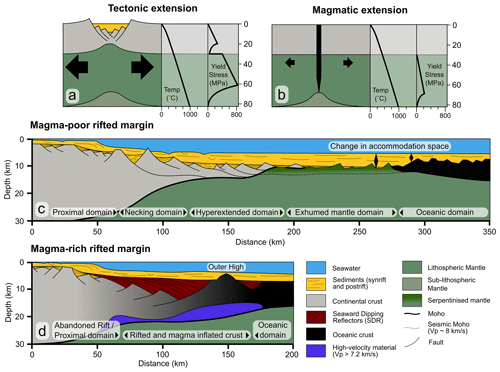
Figure 5Differences between magma-rich and magma-poor systems. (a, b) Continental rifting with and without contemporaneous magmatic intrusions, redrawn from Buck (2006). The stress required for extension to progress is significantly lower where magmatic injections weaken the lithosphere. (c, d) Characteristics of magma-poor and magma-rich rifted margins, which form during advanced rifting (break-up) with and without contemporaneous magmatism. Modified after Franke (2013).
As magma-rich systems reach their break-up configuration (Stage 3), they typically develop large-scale lava flow complexes that dip seaward, which stand out on seismic lines (hence the term seaward-dipping reflector or SDR), accompanied by continent-ward-dipping normal faults (Franke, 2013) (Fig. 5). The loading and flexure induced by these lava flows and intrusions may cause the general curved seaward-dipping nature of the SDRs and the continent-ward-dipping normal faults observed in such systems (Wolfenden et al., 2005; Buck, 2017).
2.5 Erosion and sedimentation
As rifts evolve, erosion and sedimentation (surface processes) actively shape their surface features. The associated transport and redistribution of lithospheric material can, in turn, influence large-scale tectonic processes by sedimentary loading in the basins and unloading of the eroding highs (Fig. 6; Burov and Cloetingh, 1997). Furthermore, the deposition of evaporites enables complex salt tectonic deformation (Fig. 7), and surface processes can even modify the thermal profile of the lithosphere. The expression of surface processes in rift systems is moreover impacted by climate factors.
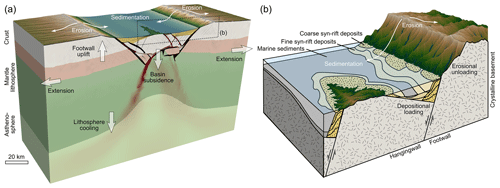
Figure 6Surface processes and tectonic deformation. (a) Lithospheric thinning leads to isostatic subsidence of the surface, which creates accommodation space for sediments. At the same time, flexural uplift of rift shoulders generates steep erodible slopes that provide a source of clastic sediments. Image based on coupled numerical models of geodynamics and landscape evolution (Neuharth et al., 2022). (b) Conceptual model of a deep syn-rift basin. Surface processes feed back on tectonic deformation by unloading the footwall via erosion while simultaneously loading the hanging wall through sedimentation. Sediment grain size generally decreases with distance from the coast but is highly susceptible to changes in sea level, tectonics, and river network dynamics. Grain size exerts first-order control over rock permeability and fluid circulation, key factors during the formation of geo-resources (Sect. 4). Image inspired by Gawthorpe and Leeder (2000).
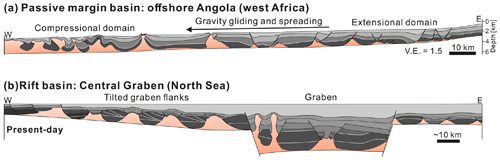
Figure 7Examples of salt tectonics from the Angolan continental margin and the North Sea Central Graben. The salt (evaporite) layer is indicated in pink. Adopted from Warsitzka et al. (2021).
Erosion of rift shoulders and other highs in a rift system removes the shallower units and exposes underlying rock units, especially when deep river incisions occur such as those of the Blue Nile on the Ethiopian Plateau (Ismail and Abdelsalam, 2012). Sedimentary loading in rift basins induces enhanced subsidence of the graben valley floor, causing large normal faults to remain active for longer (Olive et al., 2014; Neuharth et al., 2022), and can induce ductile flow in the lower crust in relatively hot rift settings (e.g. Clift et al., 2015). The stage of rifting, location in the rift basin, and the provenance of the sediments largely determine the sedimentary infill of a given site, which nevertheless may record lower-order (minor) regressive episodes during periods of falling sea level or high relative sedimentation and subsidence rates (Martins-Neto and Catuneanu, 2010). Stretching (Stage 1) sedimentation is likely continental or lacustrine, with coarse-grained deposits close to exposed fault scarps. During necking (Stage 2), quick deepening and drowning of isolated rift basins is likely to occur, with fine-grained and anoxic sediments (including potential hydrocarbon source rocks; see Sect. 4.2.1) being dominant in the basins. Fine-grained deposits continue to dominate the system during break-up (Stage 3) and drifting (Stage 4). Typically, a break-up unconformity forms, marking the rearrangement of the system during Stage 3 (Chenin et al., 2015; Morley, 2016).
The large-scale deposition of evaporite bodies in rift systems generally occurs during necking or break-up, where the former tends to result in restricted evaporite basins, whereas the latter more likely generates large and continuous evaporite deposits (e.g. along the South Atlantic margins, the Gulf of Mexico, and the Red Sea; Rowan, 2014; Augustin et al., 2014). However, some large-scale salt deposits were formed in pre-rift (Stage 0) times, e.g. the Zechstein deposits in NW Europe which were present prior to Mesozoic rifting (Littke et al., 2008), or during the stretching stage, e.g. along the Iberian and Newfoundland margins (Rowan, 2014). Due to the relative weakness of these evaporite deposits, they can be easily deformed in a ductile fashion, leading to salt tectonics (Fig. 7). Such salt tectonic deformation along rifted margins is driven by margin tilting due to thermal sag, sedimentary loading, or a combination of both, leading to highly complex halokinetic structures (Peel, 2014). In systems with pre-rift evaporite basin development, subsequent salt tectonic deformation is driven by both normal faulting in the basement and basement tilting (Warsitzka et al., 2021). It may be noted that the presence of relatively weak shale deposits can also lead to similar deformation styles to those found in salt tectonic settings (e.g. in the Niger Delta; Wiener et al., 2011).
Furthermore, the sedimentary infill of rift basins can have a blanketing effect when this infill has a low heat conductivity, leading to a general increase in temperature in the system (Freymark et al., 2017). In turn, this temperature increase can affect the rheology of the lithospheric layers, potentially increasing decoupling by lowering the strength of ductile layers (e.g. Andrés-Martínez et al., 2019). Sedimentation can also have the opposite effect via the influx of large amounts of relatively cold material (Wangen, 1995) or deposition of material with a high heat conductivity (e.g. evaporites; Duffy et al., 2023). The general influx of sedimentary infill can also partially restore the integrity of the crustal layers and may even delay continental break-up, as seen in the Gulf of California (Bialas and Buck, 2009).
Finally, climate is known to influence rift systems by controlling the rates of denudation and erosion of evolving basins in time and space (Friedmann and Burbank, 1995; Leeder et al., 1998; Salgado et al., 2016; McNeill et al., 2019). The most pronounced effect of climate on rift basins is often recorded in the type and volume of sediment deposited in the basin per se, as depositional facies and sediment influx rates vary dramatically with changing environmental conditions. One of the best examples may be the onset of microbial carbonate deposition in hypersaline basins, subject to extreme evaporation conditions, in the South Atlantic Ocean during break-up (Alves et al., 2020). However, to discern tectonic “pulses” from climatic ones can be challenging as the rift basin, sea/base level, and surrounding topography are naturally active and variable (Gallagher et al., 1999). Hence, climate change can usually be identified when analysing first- and second-order depositional sequences from different rift basins across the world, but it is not resolved easily at the third order due to the potential impact of global events suppressing local climatic signals (Mackay, 2007; Mazzini et al., 2023).
A number of natural hazards related to seismicity, volcanism, and mass-wasting processes can occur during the evolution of the various rifting stages. In this section we describe the different types of natural hazards in rift systems; their origin, frequency, and scale; the risk they pose (i.e. their potential impact on human society); and present-day mitigation options.
3.1 Seismicity
Wherever active tectonic deformation is occurring, earthquakes are likely to occur as well (Fig. 8). As such, seismicity as the result of sudden displacement along faults in the brittle parts of the lithosphere is to be expected during the whole evolution of rift systems. Although natural seismicity is an integral part of rifting and continental break-up processes, the magnitude of rift-related earthquakes remains often limited to ca. MW 7 (e.g. Yang and Chen, 2010), in contrast to the mega-earthquakes of MW>9 recorded in subduction zones (Fig. 8). This discrepancy exists because large earthquakes occur where there is a long and deep fault plane along which the rupture can propagate undisturbed, such as along a subduction interface (Thingbaijam et al., 2017). However, in rift systems, fault planes do not reach as deep as in subduction zones because of a shallower brittle–ductile transition caused by relatively higher temperatures and due to other factors such as lower pore fluid pressures. Fault networks in rifts are also less well-ordered because they accumulate significantly less strain when compared to subduction zones. This means there are more disturbances that possibly stop rupture propagation. Finally, it generally takes less stress build-up to cause displacement in rift systems, relative to compressional systems (Neely and Stein, 2021). As a result, less stress build-up and subsequent stress release in the form of large earthquakes is possible in rift systems. Even so, smaller earthquake magnitudes can still have devastating effects, as shown by various events in eastern Africa (e.g. Yang and Chen, 2010; Craig et al., 2011, and references therein).
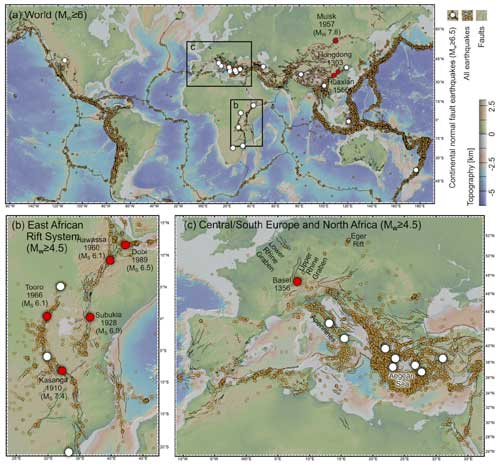
Figure 8Distribution of seismicity on a global and regional scale. Crustal normal-fault earthquakes with magnitudes MW≥6.5 are shown as white circles and have been extracted from the GCMT catalogue (time range 1976–2019; https://www.globalcmt.org/CMTsearch.html, last access: 11 July 2024), based on Neely and Stein (2021). Major rift-related earthquakes referred to in the text are shown as red circles along with their magnitudes, unless these are not well-known. All normal, thrust, and strike-slip earthquakes with MW≥6 (a) and MW≥4.5 (b, c) are depicted as small orange circles and are derived from the USGS-ANSS catalogue (time range 1960–2023; https://earthquake.usgs.gov/earthquakes/, last access: 11 July 2024). Images have been created with GeoMapApp version 3.6.14 (https://www.geomapapp.org, last access: 11 July 2024) and include topography data of Ryan et al. (2009). Fault traces are shown as black lines and are based on the GEM Global Active Faults Database (Styron and Pagani, 2020).
3.1.1 Epicentres of rifting-related seismicity
Low extension rates in continental rifts lead to very long recurrence times of large earthquakes, which impede detailed seismic hazard analysis. It is, however, clear that rift earthquakes can be devastating, as, for instance, in the East African Rift System (Fig. 8b; Ambraseys and Adams, 1991; Midzi et al., 1999). The MS 7.4 Kasanga earthquake, one of the largest ever recorded in Africa, rocked the Rukwa Rift area in 1910 (Ambraseys and Adams, 1991) and caused submarine slumps that broke telegraph cables off the coast of Mozambique (see also Sect. 3.3.2). Casualties were limited, though, most likely due to the style of local buildings at the time (Ambraseys and Adams, 1991). Other notable earthquakes with M>6 in the last century include the 1928 Subukia earthquake in the Kenya Rift (MS=6.9); the 1966 Toro earthquake in Uganda (MS=6.1); and the 1960 Hawassa earthquake (MS=6.1) and the 1989 Dobi graben event (MS=6.5), both in Ethiopia (Midzi et al., 1999; Zielke and Strecker, 2009). The Afar Triple Junction region is particularly earthquake-prone (Gouin, 1979; Goitom et al., 2017).
Significant continental rift-related seismicity is also found outside Africa. In western Europe, the year 1356 saw the total destruction of medieval Basel, near the southern tip of the Upper Rhine Graben (Meghraoui et al., 2001; Fig. 8c). Smaller earthquakes also occur regularly along the Lower Rhine Graben in Germany and the Benelux (e.g. Camelbeeck and Eck, 1994). The Aegean back-arc system is prone to rift-related quakes as well, from the Corinth rift and the area further east around Athens to the western coast of Turkey (e.g. Akçar et al., 2012; Kapetanidis et al., 2020). Other examples of active continental rift systems posing hazards are the Gulf of Suez rift (Mohamed and Abd El-Aal, 2018) and the Trans-Mexican Volcanic Belt, the latter of which contains various major cities such as Mexico City (Maestrelli et al., 2020, and references therein). The Siberian Baikal Rift system is also seismically active, with many earthquakes exceeding magnitudes of 6. This is due to the fact that the rift is situated in a cold lithosphere, where the brittle–ductile transition is deep and fault planes have a larger extent. The largest recorded Baikal Rift earthquake even reached a magnitude of MW=7.8 (the 1957 Muisk event; Doser, 1991), However, as population densities are low in the area, risks are moderate (Arzhannikova et al., 2023). Conversely, in China, exceptionally strong and deadly rift-related earthquakes are known to occur in the Shanxi Rift, southwest of Beijing (Xu et al., 2018). This rift, which has been active since the Pliocene, was the site of the 1303 Hongdong and the 1556 Huaxian earthquakes, with magnitudes of MW 8 or higher, which caused over 470 000 and 830 000 fatalities, respectively (Liu et al., 2007).
Numerous earthquakes are recorded at mid-oceanic ridges (Fig. 8), yet these pose only limited hazards due to their distance from population centres. There are situations where oceanic ridges are close to continents, or enter into continental lithosphere, and where significant earthquakes occur, such as in the Gulf of California (Castro et al., 2021) or the northern tip of the Red Sea (Hosny et al., 2013). A special case is found in southern Iceland, where the emerging Mid-Atlantic Ridge runs close to the area around the capital of Reykjavik and where historic earthquakes, such as the MS=6.0 event in 1706, have caused death and destruction (e.g. Frímann, 2011).
3.1.2 Intra-plate earthquakes
The above examples concern actively deforming rift systems. However, rift-related intra-plate earthquakes can also occur without active plate tectonic motion, i.e. in stable continental regions away from active plate boundaries that have not seen significant deformation over the recent geological past (Schulte and Mooney, 2005). A notorious example is the New Madrid Seismic Zone in the central USA, which represents a concentration of seismicity linked to the tectonically inactive Palaeozoic Reelfoot Rift and was the locus of the devastating 1811–1812 earthquakes with magnitudes over MW 7 (Calais et al., 2010). Other examples include the 1663 Charlevoix earthquake along the St. Lawrence rift zone in Quebec and the 1845 and 1888 earthquakes in the Río de la Plata region, Argentina, which were linked to the inactive Mesozoic Quilmes Trough (Rossello et al., 2020). Given that large swathes of the Earth's continental lithosphere are under some level of stress (World Stress Map; Heidbach et al., 2018), and that inactive rift systems may act as inherited weakness zones and are widely distributed (Şengör and Natal'in, 2001, Fig. 2), large earthquakes may occur at unexpected locations (e.g. Liu et al., 2007).
Furthermore, intra-plate earthquakes can strike along rifted margins, which are often somewhat misleadingly referred to as “passive margins” (see Sect. 2.1). Ongoing deformation caused by various processes, such as magmatic underplating, plate convergence, or glacial rebound, can trigger the reactivation of former rift faults to generate significant earthquake activity. A notorious example is the magnitude 7.2 Grand Banks earthquake offshore Newfoundland in 1927 (Fine et al., 2005), which caused a large submarine landslide and an associated tsunami (see Sect. 3.3.2).
3.1.3 Seismic hazard
Research and monitoring allow us to assess hazards, vulnerability, and risk in specific rift systems. Seismic surveys and geophysical analyses, satellite-imagery-based InSAR studies, and measurements of the state of stress in boreholes provide insights into the local geological setting and the dynamic deformation causing earthquakes (Illsley-Kemp et al., 2018; La Rosa et al., 2019; Heidbach et al., 2018). Important constraints on earthquake recurrence time can be deduced from historical literature (e.g. Gouin, 1979), from dating of known earthquake-prone faults by investigating sediment deposits adjacent to faults and palaeoseismic trenching analysis (Papanastassiou et al., 2005, and references therein), from directly dating the fault surface with cosmogenic nuclide analysis (e.g. Akçar et al., 2012), or by archeological research (Ambraseys, 2006; Aydan and Kumsar, 2015). Relevant data are collected and made available by various organisations, such as the Malawi Seismogenic Source Model in the East African Rift System (Williams et al., 2022), the EU-funded EPOS Seismology Consortium, and the European Facilities for Earthquake Hazard and Risk (EFEHR) Consortium, or on a global scale by the Global Earthquake Model (GEM) project. These datasets are crucial for disaster management planning and for the safe development of specific industries, especially those involving nuclear power production and fluid injection (geothermal energy projects and unconventional hydrocarbon production). For instance, the geothermal fluid injection tests in Basel, at the southern tip of the Upper Rhine Graben, caused slip along pre-stressed faults and minor earthquake damage in the city centre (Mukuhira et al., 2008). Moreover, extensive unconventional hydrocarbon production from sedimentary deposits in a variety of inactive (rift) basins in the USA causes regular seismicity with magnitudes over 5.5 MW (e.g. van der Elst et al., 2013; Chen et al., 2017).
3.2 Magmatism
There is a clear link between rift development and the occurrence of magmatic activity, and, even in so-called magma-poor rift systems, magmatism is present to some extent (see also Sect. 2.4). Magmatism in rifts dominantly occurs due to decompression melting, as the fast-upwelling mantle rocks cause temperatures to cross the peridotite solidus or the solidus of the overlying crustal rocks. This rise in hot material below a rift system can be achieved by either having a mantle plume actively pushing upward or by rapid thinning of the lithosphere. The bulk of magmatic material in rift settings remains trapped underneath the crust in the form of underplating and/or magmatic intrusions. As such, the main hazard posed by rift-related magmatism is linked to the magmatic material that reaches the surface as volcanic eruptions (i.e. causing lava flows, pyroclastic flows, ejecta, lahars/mudflows, earthquakes, and even tsunamis).
3.2.1 Hotspots of rift-related volcanism
Volcanic hazards generate the most risk in rift systems prior to the break-up stage, when the system evolves mostly in subaerial conditions, and where the fertile volcanic soils provide excellent farmland to sustain large human populations. The most prominent examples of such rift-related volcanism may be found along the highly magmatic eastern branch of the East African Rift, where, among others, Mount Kilimanjaro is situated (Martin-Jones et al., 2020) (Fig. 9). In this context, the Afar Rift at the northernmost end of the eastern branch is a special case, as some authors characterise it as an embryonic (subaerial) mid-oceanic ridge (Rime et al., 2023). This highly volcanically active region is mostly a desert at present, with parts situated down to 160 m below sea level (Rime et al., 2023), but contains considerable populations that live with the risks of frequent volcanic eruptions. For instance, although the 2008 Alu-Dalafilla eruption did not cause any human or economic losses due to the remote location of the volcano (Pagli et al., 2012), the 2011 eruption of the Nabro volcano caused several fatalities (Goitom et al., 2015). Moreover, the 2011 Nabro eruption resulted in the expulsion of 1.5 Mt of SO2, ranking it as the largest eruption since Mount Pinatubo's in 1991 (Bourassa et al., 2012), and disrupted air travel in the region (Sawamura et al., 2012). The western branch of the East African Rift System also has a moderate amount of magmatism, specifically along the linkage zones between individual rift segments that allow easier fluid migration (e.g. Corti et al., 2004; Fig. 9). As large populations are situated along the shores of the various big lakes of the western branch of the East African Rift System, the risk posed by volcanism is significant (e.g. Biggs et al., 2021; Hearn, 2022). A recent example is the 2002 devastation of the city of Goma in the Democratic Republic of Congo by a volcanic eruption of the nearby Mount Nyiragongo (Pouclet and Bram, 2021).
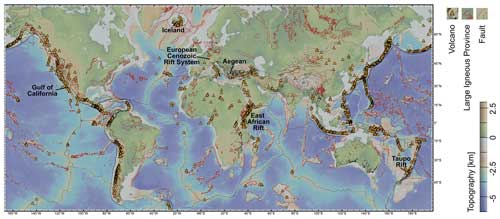
Figure 9Volcanism around the globe, based on a global catalogue of predominantly onshore volcanoes of the Global Volcanism Program (Venzke, 2023), large igneous provinces (Johansson et al., 2018), and faults and plate boundaries (Styron and Pagani, 2020). Image has been created via GeoMapApp version 3.6.14 (https://www.geomapapp.org, last access: 11 July 2024) based on topography data of Ryan et al. (2009).
The Aegean back-arc system is another location that is prone to continental volcanism, with the 1600 BCE Thera eruption on the modern-day Greek island of Santorini being perhaps the most notorious volcanic event in the recent geological past of the Mediterranean. This especially violent eruption, one of the largest in recent human history, devastated the island and sent tsunamis throughout the eastern Mediterranean (Karstens et al., 2023), and is thought to have been instrumental in the collapse of the previously flourishing Minoan civilisation on nearby Crete (Novikova et al., 2011, and references therein). The Trans-Mexican Volcanic Belt and its numerous volcanic eruptions also pose serious hazards to the various major cities in the area, including Mexico City (Maestrelli et al., 2020, and references therein). The Taupō Rift Zone, where the Havre Trough back-arc rift system enters the continental lithosphere of New Zealand, is another site of ongoing volcanism that witnessed a super-eruption as recently as 26.5 ka (Wilson et al., 2006). Other volcanic zones in continental rift settings are the Eifel, Massif Central, and Eger Graben areas of the European Cenozoic Rift System, which are less known due to the absence of present-day eruptive activity (Fig. 9). However, the last eruption in the Massif Central occurred only around 8 ka (Merle et al., 2023), whereas large-scale volcanism in the Eifel area at the northern tip of the Upper Rhine Graben lasted until some 10 ka (Dahm et al., 2020). Also, the Eger Graben further to the east exhibits geologically recent volcanic activity (Hrubcová et al., 2017). A caveat is that this rift-related volcanism in western Europe was possibly caused by mantle activity induced by the Alpine orogeny, the tectonic event that is thought to have induced the rifting in western Europe in the first place (Dèzes et al., 2004; Merle et al., 2023). Even so, there is potential for future volcanism in these areas (Boudoire et al., 2023).
After the establishment of continental break-up, oceanic rift systems tend to be submerged, which reduces volcanic hazards and their impact. Only when a submerged spreading axis is close to the continent, as in the case of the Gulf of California, could there be some risk of an eruption affecting human populations. However, in specific locations, spreading ridges (or spreading-ridge-related volcanoes) are subareal and inhabited. Examples are the volcanic Azores islands on the triple junction between the North American, European, and African plates and, most famously, Iceland, situated on the Mid-Atlantic Ridge (Gudmundsson, 2007) and site of the 2010 Eyjafjallajökull eruption that caused massive disruptions to global air travel (Gudmundsson et al., 2012; Kelman et al., 2023). Next to typical hazards experienced by inhabitants of volcanic islands such as the Azores, the population of Iceland also has to contend with eruptive melting of glaciers sitting on top of their island's volcanoes causing truly massive flash floods that devastate anything in their path (so-called jökulhlaups; Pagneux et al., 2015). Interestingly, the growth and decline of glaciers on Iceland is linked to the intensity of volcanic activity, as the associated increase and decrease in glacial load reduces or enhances decompression melting (Cooper et al., 2020). A similar correlation between glacial extent and volcanism has also been noted in western Europe (Nowell et al., 2006).
3.2.2 Volcanic degassing
In addition to the direct volcanism-related hazards such as explosive eruptions, magma-induced earthquakes, lava flows, pyroclastic flows, and lahars/mudflows that can lay waste to large swatches of land, magmatic activity in rift settings is accompanied by substantial hydrothermal circulation and degassing (e.g. Sawyer et al., 2008). These can lead to the development of mud volcanoes and geothermal fields that can pose serious hazards to local populations (e.g. Vereb et al., 2020). Hydrothermal flow can alter the state of stress in the subsurface, causing the activation of faults, and degassing can pose major local threats as well, in particular when it comes to CO2; i.e. being heavier than air and scentless, CO2 can accumulate in depressions and suffocate unaware people and livestock (Cantrell and Young, 2009). Furthermore, CO2 (and other gases) can accumulate in vast volumes in the deeper parts of the water column of lakes along rift systems. These dissolved gases in such meromictic lakes may violently escape when the delicate equilibrium in the water column is disturbed due to an earthquake, a landslide, or some (minor) eruptive activity, or possibly even without external trigger at all (Schmid et al., 2002; Tassi and Rouwet, 2014). The CO2 released during such limnic eruptions may then spread like an invisible wave through the surroundings, suffocating anyone in its path (Gusiakov, 2014). A tragic example occurred at Lake Nyos, one of a series of volcanic lakes situated along the Central African Shear Zone in Cameroon, where over 1700 people perished in 1986 (Gusiakov, 2014). Similar dangers may be lurking in the great lakes of the East African Rift System, especially as these lakes are much larger than those in Cameroon. A lake of particular concern is Lake Kivu, with millions of people living in its vicinity (e.g. Jones, 2021).
3.2.3 Mitigating volcanic hazards
Magmatism in rifts poses severe risks, but these can be mitigated to some degree. Induced degassing of meromictic lakes such as Lake Nyos and Lake Kivu reduces the risk of limnic eruptions and even allows the production of CH4 used for local energy needs (Jones, 2021; Wenz, 2020); see Sect. 4.2. While exploring for resources in such lakes, it is crucial to monitor the equilibrium in the water column so as not to accidentally cause a limnic eruption. Furthermore, there are seismic networks actively monitoring earthquakes that could kick off a limnic eruption in meromictic lakes (Oth et al., 2013). We thus have some means to actively mitigate the risk of limnic eruptions, but preventing volcanic eruptions is an unfeasible proposition, so at present we can only prepare and react when they happen. Similarly to meromictic lakes, monitoring of seismic activity, variations in degassing, or changes in ground elevation that may hint at disturbances in the magma chamber and the possibility of an imminent volcanic eruption are key monitoring methods applied in various volcanic rift settings (Biggs et al., 2021; Boudoire et al., 2023). A big challenge may be the recurrence time of eruptions in rifts that may run in the hundreds of years (e.g. Nowell et al., 2006; Einarsson et al., 2020). However, in some cases, it is possible to mitigate the potential destruction caused by lava flows by diverting their paths, as was done during the 2023 and 2024 Grindavík eruptions that threatened the important Svartsengi geothermal plant and the famous Blue Lagoon spa (Barnard, 2024). Another intriguing example occurred on the Vestmannaeyjar archipelago, just south of Iceland, where a lava flow on the main island of Heimaey in 1973 threatened to block the harbour entrance. Taking into account that fishing was the economic lifeline of the inhabitants, the lava flow was successfully prevented from advancing too far by spraying its front with large volumes of seawater (Williams and Moore, 1976).
3.3 Mass wasting
3.3.1 Subareal settings
Rifting causes the development of topography and thus of unstable slopes, either subareal or submarine, that can collapse in mass-wasting events. In subareal situations, footwall uplift of major normal faults and subsequent erosion can form impressive escarpments, such as those along the East African Rift System and the Red Sea–Gulf of Aden system, which in some cases represent some 2 km of topographic difference between rift basins and their shoulders (e.g. Fubelli and Dramis, 2015). Steep slopes and escarpments can be destabilised by mechanical weakening or by ground-shaking leading to frequent landslides (Broeckx et al., 2018). The most voluminous landslides in the East African Rift are caused by ground-shaking due to earthquakes (Jacobs et al., 2017) and by precipitation-induced weakening (Kropáček et al., 2015; Martínek et al., 2021). Particular areas at risk are the escarpments bordering the Ethiopian Highlands (Fubelli and Dramis, 2015; Martínek et al., 2021) and large parts of the western branch of the East African Rift (Stanley and Kirschbaum, 2017), for instance, along the shores of Lake Kivu (Jacobs et al., 2018; Depicker et al., 2021).
3.3.2 Submerged settings
Other, often more impactful mass-wasting events occur offshore, at the continental slopes of rifted margins. Here, large volumes of sediments accumulate over time, since the world's largest rivers drain towards rifted margins. Importantly, rifted margins in high latitudes receive major sediment input as a result of glacial erosion during ice ages. These large volumes of sediment are deposited at the angle of repose so that a slight destabilisation, either due to additional sedimentary loading, earthquakes, or sediment weakening, can cause a catastrophic collapse and massive submarine landslides. Various instances of such collapses and consequent landslides have been recorded by the severing of communication cables over the seafloor (e.g. Ambraseys and Adams, 1991; Assier-Rzadkieaicz et al., 2000). Famously, the magnitude 7.2 Grand Banks earthquake offshore Newfoundland in 1927 caused a massive submarine landslide, including turbidite flows that travelled over a distance of over 1000 km at speeds ranging between 60–100 km h−1, thereby severing telegraph cables (Fine et al., 2005). Communication cables can be replaced, but tsunamis are much more impactful hazards generated by submarine slides, such as the one that resulted from the 1927 Grand Banks earthquake, which claimed 28 victims (Løvholt et al., 2019). However, the most well-known submarine landslide is the Storegga landslide that occurred along the Norwegian rifted margin in ca. 8000 BCE (Bondevik et al., 2005). The resulting tsunami drowned the coasts of Norway, the Shetland and Orkney Islands, eastern Greenland, and northwestern Europe down to the southern North Sea (Nyland et al., 2021). Many other submarine landslides and associated tsunamis are known from the northeastern Atlantic (e.g. Leynaud et al., 2009) and from rifted margins around the globe (e.g. Korup, 2012; Thran et al., 2021; Fig. 10). Since many large cities are located along the coast near rifted margins, the impact of tsunamis induced by large submarine slides such as those offshore Norway would be catastrophic. We should, however, emphasise that the recurrence intervals for these large submarine landslides are very broad (Leynaud et al., 2009). Still, climate change and associated potential destabilisation of rifted margins increase the risk of landslide tsunamis in the North Atlantic and along other rifted margin settings.
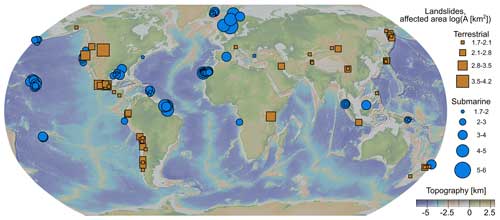
Figure 10World map of giant terrestrial (squares) and submarine (circles) landslides. Rifted margins in the North Atlantic are particularly prone to hosting large submarine landslides because they (1) accumulate large volumes of sediments and (2) experienced enhanced sediment loading during glacial periods. The size of the symbols corresponds to the landslide surface area. Modified from Korup (2012).
Rift systems contain a wealth of geo-resources that will be greatly needed for the energy transition and the establishment of a sustainable economy in the 21st century. In this section we describe (1) the non-energy mineral resources, (2) the various geo-energy resources, and (3) the temporary and permanent geological subsurface storage options that occur in rift environments.
4.1 Non-energy mineral resources
4.1.1 Mineral deposits
Mineral deposits are anomalous concentrations of minerals in rocks or sediments, while ore deposits are those deposits that are economically viable to extract (Heinrich and Candela, 2014). The mineral deposits related to rift systems can be divided into various categories depending on when and how they formed (e.g. Groves and Bierlein, 2007; Zappettini et al., 2017; Fig. 11).
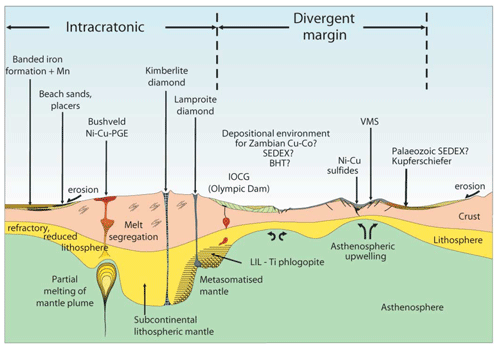
Figure 11Schematic diagram of major mineral deposit types formed in continental crust and along rifted margins and oceanic spreading ridges. Adopted from Groves and Bierlein (2007).
Pre-rift mineral deposits are of great importance in rift systems. They come in many shapes and forms, depending on the pre-rift (Stage 0) geological history of the specific setting. The rocks containing pre-rift deposits are exhumed by the combined action of extensional tectonics and erosion at the rift shoulders, along individual rift blocks, or along (uplifted) rifted margins. Much of the mineral wealth of the countries along the East African Rift System is based on such deposits exhumed during rifting (e.g. Taylor et al., 2009; Dill, 2007). Other examples of pre-rift deposits are those which are (historically) exploited along the Cenozoic European Rift System (e.g. the Vosges and Black Forest mountains and the Massif Central), where the Hercynian metamorphic basement is exposed (e.g. Forel et al., 2010; Steiner, 2019).
Sedimentary mineral deposits are generated by sedimentary processes. One type of sedimentary deposit, called “(palaeo)placers”, is found in clastic sedimentary environments. Placer deposits form through the breakdown of bedrock and the sorting and concentration of relatively heavy minerals, such as diamonds, gold, and platinum-group minerals, by various sedimentary processes (mostly fluvial; Ridley, 2013). Deposits thus form at shorelines and in rivers and are found, for example, in the East African Rift System and along the rifted margins of eastern Africa and the Russian Arctic (Dill and Ludwig, 2008; Bochneva et al., 2021). High-grade diamond deposits are found and exploited offshore Namibia (Schneider, 2020). Not all placer deposits are economical, but they can still provide insights into the location of their (pre-rift) origin sites through provenance analysis (Gong et al., 2021).
Hydrogenetic deposits, which are chemically precipitated from surface waters, can also be found in rift environments, for example, ancient or actively forming evaporites. Evaporites develop in compartmentalised basins through solar evaporation and can be mined to produce salt for human consumption or industrial use, as is the practice in present-day Afar and in Lake Magadi in Kenya (Kodikara et al., 2012; Varet, 2018). Depending on the origin of the salt and fluids (marine or hydrothermal, or even from serpentinisation of exhuming mantle material, referred to as “dehydrates”; Debure et al., 2019), there may also be important concentrations of potash, lithium, trona, and other minerals to be extracted (Kodikara et al., 2012; Bekele and Schmerold, 2020). For instance, phosphorites are phosphorus-rich hydrogenetic deposits that (bio)chemically form in the shallow marine environment along rifted margins (i.e. on the continental shelf; Ridley, 2013; Kudrass et al., 2017). Given the projected scarcity of phosphorus, a component of fertiliser (Alewell et al., 2020), these relict and recent offshore deposits will be of great interest to the global community in the near future.
Sediment-hosted hydrothermal ore deposits form in sedimentary rift basins both during and after rifting. Hydrothermal fluids circulating through the basins leach metals from deeper in the basin, transport them upwards, and deposit them in sulfide minerals when reaching conditions that trigger metal release (Heinrich and Candela, 2014; Wilkinson, 2014). Temperature, salinity, pH, and redox state all affect the solubility of metals in these fluids (e.g. Heinrich and Candela, 2014; Zhong et al., 2015), making heated saline waters an efficient mineralising fluid and colder, reduced rocks a possible host (Ridley, 2013; Wilkinson, 2014).
Sediment-hosted mineral deposits are the largest global resource of lead and zinc (Goodfellow et al., 1993; Mudd et al., 2017), which are both base metals with great importance to the global energy transition (Blowes et al., 2014; IEA, 2021). The majority is found in clastic-dominated (CD)-type deposits (formerly known as SEDEX deposits; Goodfellow et al., 1993) that formed during deposition or diagenesis of the marine siliciclastic host rock through subseafloor replacement of barite with lead–zinc sulfides (syngenetic to diagenetic mineralisation; Leach et al., 2010; Magnall et al., 2020). These mostly Proterozoic and Paleozoic mineralisations occur close to syn-sedimentary faults (e.g. Wilkinson, 2014; Hayward et al., 2021), which could have provided permeable pathways focusing upward fluid flow (e.g. Walsh et al., 2018; Sheldon et al., 2019; Rodríguez et al., 2021). Large deposits have been found in the Carpentaria Zinc Belt in Australia, the North American Cordillera, Russia, and China (e.g. Leach et al., 2010; Hoggard et al., 2020). The precursory sediment-hosted barite deposits are also mined in China, India, and the USA (e.g. Clark et al., 2004).
Contrary to CD-type deposits, Mississippi Valley-type (MVT) lead–zinc deposits formed much later than their host rock (up to tens of millions of years; epigenetic mineralisation). The mostly Phanerozoic MVT deposits are found in broad rift basins with thick sequences of shallow marine sediments including platform carbonates that host the mineralisation (Ridley, 2013). These basins have generally been affected by inversion (e.g. Leach et al., 2010), with high topography and/or tectonic loading possibly having driven long-distance fluid flow (Ridley, 2013). By finding correlations between geophysical and geological observational datasets and the locations of known CD-type and MVT deposits, researchers try to limit the exploration space for these deposits (e.g. Hoggard et al., 2020; Lawley et al., 2022; Burisch et al., 2022). For example, Hoggard et al. (2020) found that all giant deposits lie within 200 km of the edges of cratonic lithosphere.
A big supplier of copper is the sediment-hosted stratiform copper (and cobalt) deposits as found in the Kupferschiefer field in the Zechstein Basin (Poland and Germany), the Central African Copperbelt, the Kodar–Udokan basin in Siberia, and the White Pine deposit in the USA. These deposits mostly occur at stratigraphic and redox boundaries between terrestrial syn-rift redbed sandstones (metal source) and overlying shallow-marine or lacustrine organic-rich shales (host rock; Hitzman et al., 2010; Ridley, 2013). Such successions formed in the intracontinental basins of failed rifts, with syn- or post-diagenetic mineralisation by oxidised saline fluids during the sag or even the inversion phase (Brown, 2014; Hitzman et al., 2010).
Intracontinental basins also contain several types of sediment-hosted uranium deposits (e.g. Robb, 2004; Dahlkamp, 2009). For example, epigenetic-unconformity-related deposits (such as in the Athabasca Basin, Canada; Jefferson and Delaney, 2007) are found at major unconformities between the basement and overlying redbeds (Dahlkamp, 2009). Tabular and roll-front deposits have distinct blanket and U-shaped geometries, respectively, and are formed by lower-temperature oxidised fluid circulating in the sandstones (Ridley, 2013).
An example of magma-related hydrothermal ore deposits, or volcanic-hosted or volcanogenic massive sulfide (VHMS or VMS) deposits, formed and currently form through venting of hydrothermal fluids in and at the seafloor (creating, for example, black and white smokers; Barrie and Hannington, 1997; Hannington et al., 2005). The first seafloor hydrothermal activity was discovered in the Red Sea oceanic spreading centre (Miller et al., 1966), and many more sites have been identified since. The majority of active VMS deposits occur along mid-oceanic ridges, where mostly seawater circulates in the subsurface, depositing polymetallic sulfide minerals that contain, amongst others, copper, zinc, lead, gold, and silver (e.g. Shanks et al., 2012; Fuchs et al., 2019). Some fossil VMS systems are found exposed after being obducted by subsequent convergence tectonics. The Oman ophiolite is a well-known example, but the Troodos Ophiolite on Cyprus is world-famous since its VMS deposits have been exploited for thousands of years to the degree that copper (cuprum, in Latin) may have been named after the island (Pirajno et al., 2020). Currently active VMS systems are common along mid-oceanic ridges but are also found in the (incipient) spreading centres of Afar (e.g. at Dallol; Varet, 2018).
Diamondiferous kimberlites are a well-known type of magmatic ore deposit that contain diamonds. Kimberlites are rocks formed from highly volatile ultramafic eruptions in cratons, shields, and mobile belts (Jelsma et al., 2009), which can transport diamonds that are formed at large depths within the mantle (>150 km) to the surface (Ridley, 2013). Recently, Gernon et al. (2023) correlated kimberlites with periods of dispersal of the continental plates, with a lag of about 30 Myr between break-up and kimberlite volcanism. Also, the emplacement of the Argyle lamproite diamond deposit in the intracontinental rift in the Halls Creek Orogen (Australia) was probably driven by the break-up of the Nuna supercontinent (Olierook et al., 2023). Other magmatic ore deposits linked to rifting are layered intrusions, such as those of the Bushveld Complex in the Kaapvaal Craton, which likely formed in a back-arc setting and contain, amongst others, copper, nickel, and platinum-group metal deposits (Clarke et al., 2009) (Fig. 11). Moreover, syn-rift carbonatites, found, for example, along the East African Rift System and in the Bayan Obo deposit in China, produce the majority of rare-earth elements and niobium (Smith et al., 2015; Yaxley et al., 2022). Carbonatite volcanism is rather rare in the geological record, and the Ol Doinyo Lengai in the Tanzanian part of the East African Rift System is the only currently active volcano that produces carbonatites (Kamenetsky et al., 2021). Chromite can be found both in layered intrusions like the Bushveld Complex (e.g. Mondal and Mathez, 2007) and in mid-oceanic-ridge and back-arc ophiolites (e.g. the Troodos, Oman, and Kempirsai ophiolites; Melcher et al., 1997; Ahmed and Arai, 2003; Pirajno et al., 2020).
4.1.2 Aggregate (construction) materials
Rift environments contain a variety of aggregate mineral resources that can serve as construction materials. For instance, deposits of sand and gravel in rift environments are of great importance for construction projects, since they are used for roads and building foundations and for the preparation of cement and concrete (Hearn, 2022). Clay deposits can be used for brick production, and sedimentary rocks are used for the construction and decoration of buildings. For instance, Triassic Buntsandstein outcropping on the rift shoulders of the Upper Rhine Graben has always been a popular building material in the region (Heap et al., 2017). Another application is the use of Kieselkalk, which was deposited on the European rifted margin prior to the Alpine orogeny (https://www.strati.ch, last access: 11 July 2024) as railway track ballast (Suhr and Six, 2020). Volcanic deposits found in rift settings are also of interest to construction. Imported basalt blocks have been applied to reinforce waterworks in the Netherlands (Wichman et al., 2009), and volcanic rocks were used to construct the old town of Clermont-Ferrand and its iconic black cathedral in the Massif Central (Dompnier et al., 2014). Eruptive materials in the East African Rift System are used for various local construction purposes but have the drawback that their characteristics are rather unpredictable due to their heterogeneous composition and grain size variations (Walle et al., 2000; Hearn, 2022). Even so, cinder material is a reasonable substitute for scarce gravel in eastern Africa (Hearn, 2022).
4.1.3 Helium gas
Another highly valuable resource that can be produced in rift systems is helium gas, which is used for a wide range of applications ranging from healthcare to space flight (National Research Council, 2010). A highly strategic non-renewable resource, helium is normally extracted from rocks in felsic cratons, which accumulate the gas that is generated as a by-product of uranium and thorium decay (Hand, 2016). However, unusually high helium fluxes in the Tanzanian part of the East African Rift System have recently caught the attention of exploration geologists, and large helium reservoirs have been discovered (Danabalan et al., 2022). The cause of these high fluxes is the increased heat of the rift system, which releases the helium from cratonic rocks, after which it accumulates in porous reservoir rocks from which it can readily be produced (Hand, 2016).
4.2 Geo-energy resources
Geo-energy resources come in various forms. Most well-known are hydrocarbons or fossil fuels (petroleum, natural gas, and coal), which have fuelled our economies since the industrial revolution and allow our present-day living standards. However, the use of hydrocarbons has caused massive greenhouse gas emissions, leading to increasingly severe climate change (e.g. IPCC, 2023). Consequently, one of the biggest challenges for the energy transition will be to find new energy sources to replace hydrocarbons and reduce greenhouse gas emissions. Two promising geo-energy sources that can be produced in rift environments are natural hydrogen gas (H2) and geothermal energy.
4.2.1 Hydrocarbons and fossil fuels
Hydrocarbons presently represent the most well-known energy resources produced in rift systems, with massive oil and natural gas deposits found in petroleum provinces such as those in the North Sea rift and UK–Norwegian margin and in the Gulf of Mexico (e.g. Levell and Bowman, 2018; Snedden and Galloway, 2019; Fig. 12). Rift systems provide ideal environments for the development of conventional petroleum systems. Firstly, they allow the development of hydrocarbon source rocks, typically fine-grained marine or lacustrine sediments rich in organic material, in restricted rift basins with fast subsidence, limited water circulation, and low O2 content, so that the organic matter is preserved and only minor amounts of other sediments are deposited in these sediment-starved environments (Katz, 1995; Nemčok, 2016). Rapid subsidence in rift basins also allows the source rock to be buried to a depth of 2–7 km by subsequent sedimentary infill to reach temperatures of ca. 100–250 °C that allow the generation of oil and natural gas (Nemčok, 2016). Furthermore, the large variety of deposits in rift systems ensures that there is a high likelihood that reservoir rocks are available, especially in earlier rift stages and near active normal faults (Fig. 6; White et al., 1999; Gawthorpe and Leeder, 2000). The upward migration of buoyant hydrocarbons from the source rock into reservoir rocks can occur vertically, but pathways such as high-permeability sedimentary rock layers and normal faults in rift systems, in particular where individual rift basins merge, allow enhanced hydrocarbon migration (Fossen et al., 2010). The presence of impermeable rock layers, such as clays (or evaporites) that generally develop in the later stages of rifting, act as seals or cap rocks, forcing hydrocarbons to migrate around them (de Jager and Geluk, 2007). However, when this is not possible, as in the case of a tectonically or stratigraphically formed trap structure, a hydrocarbon field can develop, which can be drilled and exploited (Nemčok, 2016). It is key that these elements need to be present at the right time and the right place, so a thorough understanding of a basin's geological history is required to successfully exploit its conventional petroleum system(s) (Magoon and Dow, 1994; Alves et al., 2020).
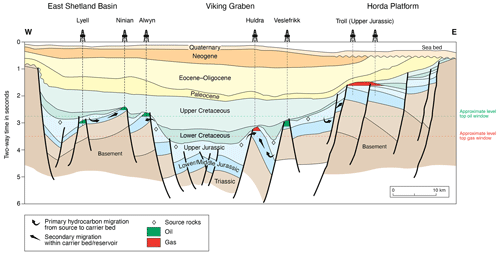
Figure 12Petroleum system in the northern part of the Mesozoic North Sea rift system. Charge is from the syn-rift Upper Jurassic source rocks, migration follows faults and permeable layers, the main reservoir/seal pair is the Middle Jurassic pre-rift Brent Formation. The petroleum traps are tilted footwall closures below the overlying Lower Cretaceous shales. After Husmo et al. (2003).
Furthermore, relatively recent technological developments have allowed the exploitation of unconventional petroleum systems, which include shale oil and/or shale gas and are much simpler than conventional petroleum systems since the source rock is simultaneously the reservoir rock (Muther et al., 2022). Large volumes of hydrocarbons remain trapped in the impermeable fine-grained source rocks themselves, and they can be released and produced by directly drilling into the source rocks followed by hydraulic stimulation or “fracking” (Bažant et al., 2014; Li et al., 2015). Rift settings provide an ideal environment for the development of source rocks, which can be exploited even when no suitable conventional reservoirs, traps, or seals are present in the system. Such unconventional production of shale oil and gas has been booming in the USA since the late 20th century, with various rift basins producing unconventional oil and gas (e.g. the Southern Oklahoma Aulacogen; Keller and Stephenson, 2007; van der Elst et al., 2013). Although there seems to be good potential in other rift basins around the globe (e.g. the North Sea rifts; the Pannonian and Donetsk basins in Europe; or the Songliao, Fuxin, and Bohai basins in China; Schulz et al., 2010; Zhang et al., 2023), these resources have not yet been developed. A notable exception is the Neuquén Basin in Argentina, which contains the highly prolific Vaca Muerta source rock that was deposited in a back-arc rift setting (Howell et al., 2005).
Gas hydrates represent another type of non-conventional hydrocarbon resource that is abundantly found along rifted margins (Ruppel and Kessler, 2017) and uniquely in the continental Baikal Rift as well (Khlystov et al., 2019). At great depth, or at low temperatures in more shallow marine environments such as in the Arctic, natural gas (commonly generated by biochemical activity in the seafloor) and water can form an ice-like compound referred to as gas hydrate (Ruppel and Kessler, 2017). Massive deposits of gas hydrates are known to exist along rifted margins, and various pilot projects have been undertaken to explore their potential (e.g. at the margin of the Canadian Arctic and the South China Sea; Yamamoto et al., 2022).
Finally, hydrocarbons also come in solid form, i.e. as coal, which stems from plant material accumulated and preserved in swamps that is subsequently buried and increasingly enriched in carbon. During this coalification process, the peat first turns into lignite (brown coal), and via a number of intermediate stages the purest type of coal (anthracite) can be formed (Diessel, 1992). Various coal fields have developed in rift settings, especially where subsidence was not too fast so that peat swamps could develop, such as in the marginal basins on both sides of the North Atlantic (Diessel, 1992). Coal layers generate natural gas that can accumulate in hydrocarbon reservoirs, but this gas can also be directly exploited, similarly to unconventional shale gas source rocks (coal-bed methane; Moore, 2012; Muther et al., 2022). Moreover, the various types of coal have been mined for a long time, fuelled the industrial revolution, and remain an indispensable energy source for many countries around the world to this day (Pudasainee et al., 2020).
4.2.2 Natural hydrogen (H2)
Hydrogen gas (H2) is a clean source of energy, as its combustion generates nothing but water as a by-product. The problem is, however, that present-day H2 production is costly at best (when using green energy) or highly polluting at worst (when using fossil energy) (e.g. Ajanovic et al., 2022; Osman et al., 2022). However, various sources of naturally occurring H2 exist, of which the most promising is the serpentinisation of (ultra)mafic rocks (e.g. mantle rocks): by reacting with water, these mantle rocks release natural H2 (Fig. 13) (e.g. Smith et al., 2005; Gaucher, 2020). Large amounts of natural H2 are released during the more advanced stages of rifting (i.e. break-up and drifting, when mantle material is being exhumed and serpentinised) (e.g. Albers et al., 2021; Liu et al., 2023; Fig. 13), and it is speculated that such natural H2 could have played a key role in the emergence of life on Earth (Russell et al., 2010). Of key importance is that water can reach down to the mantle material, for instance, via large normal faults, but complex and deep-rooted fault patterns in rift transfer and transform zones provide improved opportunities for water circulation, as is also the case for magma and hydrocarbon migration (see Sect. 4.2.1). Similarly to conventional petroleum systems, the generated natural H2 from such “hydrogen systems” will have to migrate from the (mantle) source rock to reservoirs in order to be exploited, or it may even be possible to directly drill into the mantle and hydraulically stimulate the mantle source rock in order to serpentinise it (Zwaan et al., 2023; Osselin et al., 2022). In the case of a natural H2 reservoir, the highly reactive character of H2 means that it can be lost through various (bio)chemical processes. Therefore, reservoirs will ideally have temperatures between 100–200 °C, at which H2 is relatively inert (Lefeuvre et al., 2022).
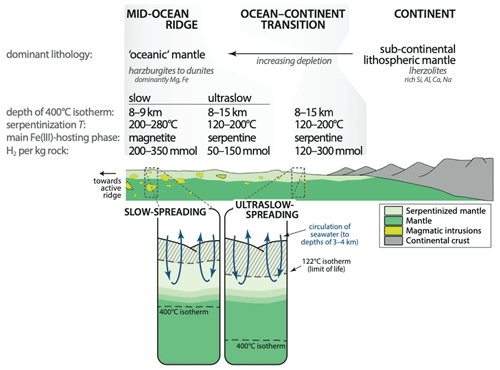
Figure 13Sketch of natural H2 generation related to serpentinisation at (magma-poor) rifted margins and along ultraslow- and slow-spreading mid-oceanic ridges. Differences in H2 generation potential are due to petrological variations in the serpentinising rocks and changes in the thermal regime, which itself strongly depends on the divergence velocity. After Albers et al. (2021).
4.2.3 Geothermal energy
Finally, geothermal energy production is a developing industry in rift basins around the world (Jolie et al., 2021). The thinning of the lithosphere and the rise of hot mantle material towards the surface create an elevated geothermal gradient, and the resulting higher heat flow can be exploited. Geothermal springs in rift basins such as the Upper Rhine Graben have been in use for bathing and healing purposes since at least Roman times (e.g. Sanner, 2000). Furthermore, warm water from shallow aquifers (low-enthalpy systems) (Lee, 2001) can be used for heating greenhouses and other buildings (Aydin and Merey, 2021), whereas water from deep aquifers is hot enough for power production (high-enthalpy systems; Stober and Bucher, 2021). The drilling targets for geothermal power production pose a challenge, as they may be situated in the deepest part of the rift basins, in contrast to drilling targets for hydrocarbon production that tend to occur higher up in the basin stratigraphy (Weert et al., 2023). However, geothermal energy production also provides additional mining opportunities by extracting dissolved elements and minerals from the geothermal fluids (e.g. lithium, rare earths, salts; Kölbel et al., 2023). Various geothermal projects are currently underway in continental rifts (e.g. in Soultz-sous-Forêts, France, and Olkaria, Kenya; Ledésert et al., 2021; IRENA, 2020), with the East African Rift as an obvious target (Martin-Jones et al., 2020). However, subareal oceanic spreading ridges in Iceland and Afar provide perhaps the greatest potential. In the Icelandic case, the country has such a massive surplus of geothermal energy that energy-intensive industries such as aluminium production have established themselves (Leifsson, 1992). The potential in Afar remains untapped as of now, but its geothermal setting, which is similar to that of Iceland, could provide the surrounding regions with copious amounts of green energy in the future (Cherkose et al., 2023).
4.3 Fresh water and fertile soils
Next to mineral and energy resources, rift environments also provide geo-resources that are crucial to sustain life, such as water and soils. Fresh water is vital to human survival but is also an indispensable resource for many industries. In rift systems, meteoric water precipitates on rift shoulders or uplifted rifted margins such as the Ethiopian Plateau, where two-thirds of the Nile water originates (Pacini and Harper, 2016), and, for instance, the coastal ranges of western India (Sharma et al., 2022) or Norway (Maystrenko et al., 2020). Infiltrating meteoric water can accumulate in porous rock layers (aquifers), from which it can be produced by drilling wells (e.g. the Kobo–Girana Valley Development Programme in Ethiopia; Zwaan et al., 2020). Fresh water also accumulates in continental rift basins, where the world's deepest lakes such as Lake Baikal in Siberia and the Great Lakes of the East African Rift System represent important reservoirs. Furthermore, offshore fresh, or relatively freshened, groundwater that is stored in rifted margin sediments has recently been identified as a potentially vast resource with a global volume of 1×106 km3. Such offshore freshened groundwater predominantly occurs within 55 km of the coastline and is thought to have been primarily emplaced during Pleistocene sea-level low-stands (Micallef et al., 2021). By contrast, some lakes found in rift settings are highly saline due to excessive evaporation, the large salt deposits in the subsurface through which groundwater flows into the lake, or partial sourcing by mineral-rich hot springs (e.g. Lake Afrera in the Afar Rift and Lake Magadi in Kenya), providing opportunities for wellness activities, salt production, and rare-element extraction (e.g. Kodikara et al., 2012; Varet, 2018; see also Sect. 4.1.1).
Highly fertile soils are another important resource that is abundant in rift environments. Such soils may come in the shape of large amounts of (fine) sediment deposits, especially in areas with large rivers and large deltas. For example, the yearly flooding of the Nile transports large volumes of silt from the Ethiopian Highlands downstream to Sudan and Egypt (Fielding et al., 2018). Individual rift basins can also accumulate large volumes of fertile sediment (e.g. along the East African Rift System or the European Cenozoic Rift System). In the case of the Nile, the silt that is dominantly brought in from the Ethiopian Highlands is extremely fertile due to its volcanic origin, since these highlands are covered by basaltic layers up to 2 km thick (Fielding et al., 2018). The presence of such rift-related volcanic rocks and soils also allows extensive agriculture in Ethiopia, supporting tens of millions of Ethiopians living in the highlands (Hurni et al., 2010). Farther south, the eastern branch of the East African Rift System is highly prone to volcanism, providing large extents of fertile soils, and similar fertile soils are also found, but to a lesser degree, along the less-volcanic western branch of the same rift system (Ebinger, 1989).
4.4 Geological storage
In the effort to achieve energy security for net-zero societies, geological storage is important for a number of reasons. Firstly, geological storage of fuels (e.g., hydrocarbons, hydrogen gas) allows the medium- to long-term accumulation of large volumes of energy-dense fluids and gases far from their location of production and near to where they will be required, improving energy security amongst seasonal changes in energy demand and uncertainties within the international energy supply chain. Moreover, the geological storage of energy (fuels, compressed air, heat) over the short to medium term aids in balancing the significant unpredictability and seasonal fluctuations inherent to renewable energy sources (e.g. wind, solar), allowing these energy sources to meet the fluctuating demands of society (Mitali et al., 2022). Secondly, geological storage provides a means to safely dispose of waste products, e.g. carbon dioxide, which require long-term isolation from the atmosphere and/or biosphere.
4.4.1 Temporary storage
The best-known temporary geological storage targets are old hydrocarbon fields and porous rock layers, which are plentiful in rift systems. For instance, in western Europe, excellent reservoirs provide temporary storage for natural gas imported from other regions of the world (Tarkowski et al., 2021), smoothing supply to meet daily to seasonal changes in production versus demand and providing grid-scale buffering for renewable energy from wind an solar sources, thus improving energy security. Porous rock layers also provide opportunities for Aquifer thermal energy storage (ATES) and high-temperature ATES (HT-ATES). Through ATES, heat can be transferred to water and injected into relatively shallow reservoir layers, where the heat can be effectively stored due to decreased heat loss due to advection (water flow) and diffusion (Fleuchaus et al., 2018). Of key importance for storage of natural gas and heat in subsurface reservoirs is their widespread availability and excellent permeability, which allows the gas or hot water to be easily injected and extracted (Tarkowski et al., 2021).
Another storage target in rift systems involves salt caverns, created by dissolution mining of salt in salt diapirs or by physical mining of evaporite layers (Duffy et al., 2023; Williams et al., 2022; Fig. 14). In contrast to porous rocks, which provide storage space between (sedimentary) grains, caverns and mine shafts are wide, open spaces in the subsurface, which allow rapid injection and extraction of liquid or gaseous resources. Salt is highly impermeable and self-sealing, and caverns provide ideal storage opportunities for strategic petroleum reserves, H2 gas, helium gas, and compressed air (Duffy et al., 2023). Such storage sites have been developed in the rifted margin of the USA Gulf of Mexico, which contains a large salt tectonic system (e.g. Sobolik et al., 2019; Tarkowski, 2019). In particular, three commercial compressed-air energy storage (CAES) facilities currently exist in Germany, the USA, and Canada, each exploiting salt caverns (Kim et al., 2023). A limiting factor for the use of salt caverns for CAES is the distribution and accessibility of suitable salt deposits, although recent work has shown that compressed air may also be stored in porous media with capacities suitable for national grid-scale energy storage and efficiencies of up to 0.67 (Gasanzade et al., 2023). However, care is required to inject compressed air into depleted oil and gas reservoirs due to the potential for a combustible environment at the surface or in the subsurface (Kim et al., 2023).
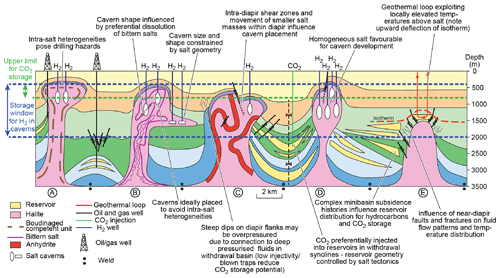
Figure 14Use of subsurface evaporite (salt) structures for various geological purposes (energy storage in salt caverns, CO2 storage, geothermal energy, oil and gas exploration). The great variety in salt diapir geometries and inter-diapir architecture of sedimentary rocks highlights that each setting is unique and requires a thorough understanding of its genetic processes in order to be successfully used. Adopted from Duffy et al. (2023).
4.4.2 Permanent storage
In our push to establish a sustainable economy, we should not only apply the resources available to us but also responsibly process waste products. Where some of these waste products can be recycled, some cannot and need to be permanently and safely stored, which can be done by making use of geological formations found in rift settings.
The most well-known waste product generated by industrial activity is CO2, which is generally released into the atmosphere, where it subsequently is one of the principal causes of global warming. As such, it is essential to reduce the atmospheric CO2 content by capturing and permanently storing it, a process commonly referred to as carbon capture and sequestration (CCS) (Tucker, 2018; Bajpai et al., 2022). For instance, CO2 can be stored in saline aquifers or depleted hydrocarbon reservoirs in rift environments (Ajayi et al., 2019), where it is simply pumped into the reservoir to take the place of the previous hydrocarbons (Tucker, 2018). Buoyant CO2 is initially trapped in the reservoir by an overlying cap rock, but with time CO2 can become more securely stored by other mechanisms such as residual trapping, dissolution, and mineralisation (Jun et al., 2017). The possibilities of applying such CO2 storage in depleted hydrocarbon fields in, for instance, the North Sea rift and along the rifted margins of the Gulf of Mexico, China, and Australia are being studied (Agartan et al., 2018; Bajpai et al., 2022), with a successful CCS project being under way in the Danish North Sea (Skopljak, 2023). However, the storage of CO2 in depleted hydrocarbon fields comes with a number of potential challenges, including leakage of the buoyant CO2 due to abandoned infrastructure (e.g. boreholes) and human-induced seismicity during injection/extraction (e.g. Ajayi et al., 2019).
In some active hydrocarbon fields, CO2 is also being pumped into the reservoir to increase its internal pressure, thus enhancing hydrocarbon production while storing CO2 (Whittaker et al., 2011; Bajpai et al., 2022), a process referred to as enhanced oil recovery (EOR) or carbon capture utilisation and sequestration (CCUS). In parallel, CO2 can also be permanently stored in sedimentary formations with poor permeability such as clay- or evaporite-rich deposits, i.e. the opposite of depleted hydrocarbon reservoirs (Duffy et al., 2023; Fig. 14). The advantage of injecting CO2 in such formations is that no reliable cap rock is needed as in a conventional reservoir, akin to the source rock in unconventional hydrocarbon systems (see Sect. 4.2.1), the impermeable formation will act as both the reservoir and the seal for the injected CO2.
Next to permanent storage in depleted porous media reservoirs and impermeable sedimentary strata, CO2 can also be chemically stored when it reacts with unaltered (fresh) exhumed rocks, a process known as carbonation (e.g. Kellogg et al., 2019). As such, researchers have proposed a link between global phases of plate-convergence-driven mountain-building and cooling climates (e.g. Kellogg et al., 2019). Fresh crustal rocks can also be exhumed during plate divergence, e.g. on the rift shoulders of continental rift systems, leading to natural carbonation and storage of atmospheric CO2. Even so, carbonation is much more efficient when (ultra)mafic rocks are available, such as mantle rocks or basalts (Matter and Kelemen, 2009). Fresh mantle rocks may be exhumed along the rift axis or spreading ridge during the break-up stage in magma-poor systems and during the drifting stage in slow-spreading systems (e.g. Albers et al., 2021; Liu et al., 2023). CO2 could be artificially injected into these exhumed mantle rocks for storage via enhanced carbonation (e.g. Olajire, 2013; Snæbjörnsdóttir et al., 2020). In magma-rich systems, flood basalts that erupted in continental settings, or extensive basalt flows forming the seaward-dipping reflectors during the break-up stage, could similarly be injected with CO2 for permanent storage (Fedorik et al., 2023; Okoko and Olaka, 2021). The advantage of injection in mafic rocks over porous media reservoirs is the enhanced potential for mineral trapping so that the CO2 will be permanently fixed to the host rock (Matter and Kelemen, 2009). Projects to explore the possibilities of injecting CO2 into basaltic rocks are under way, for instance, in Iceland (Carbfix project; Snæbjörnsdóttir et al., 2020) and in the Red Sea, offshore Saudi Arabia (Fedorik et al., 2023).
Finally, the use of nuclear power provides an alternative to burning hydrocarbons and permanently storing the resulting CO2. However, the resulting nuclear waste can remain hazardous for thousands of years, requiring its own permanent geological disposal with isolation and containment demonstrable for up to 1 million years. Therefore, suitably stable, deep (often >200 m b.s.l.), and low-permeability geological formations are required. Salt or clay formations deposited in abandoned rifts are commonly proposed as appropriate geological storage sites due to their extremely low permeabilities and self-healing properties (Turner et al., 2023).
5.1 Fundamental research
Rift research has come a long way since the days cartographers first noted that the coastlines of South America and Africa would fit together remarkably well (Romm, 1994). Our understanding has evolved from the concept of continental drift via the recognition of oceanic spreading and the occurrence of subduction to the present-day concept of plate tectonics. However, there are various important scientific questions associated with rifting that remain to be answered. A recent white paper by Peron-Pinvidic et al. (2019) sums up a number of key topics to which these questions are linked and which should be considered in a 3D framework to account for the numerous lateral variations in rift systems (Sect. 2.3) rather than the more traditional but limited 2D view.
-
Rheology. Various factors impact the rheology of the lithosphere during rifting, leading to a wide range of rift architectures (e.g. Sapin et al., 2021), but we need to better constrain the timing and interaction between these factors.
-
Inheritance. Inheritance is known to influence the localisation of deformation during rifting, but the exact influence of the different types of inheritance is challenging to entangle. Furthermore, rift inheritance can also affect subsequent contractional deformation (i.e. the “other half” of the Wilson Cycle; Fig. 1), which remains poorly understood.
-
Faults and deformation. Rifting is generally accommodated by the development of faults and shear zones, but a detailed understanding of early fault evolution remains elusive (e.g. Rotevatn et al., 2019).
-
Stratigraphy. This topic covers how sedimentary processes react to tectonic deformation, and vice versa, and how well classical models fit with new insights (e.g. Masini et al., 2013).
-
Kinematics. Rift systems can form under a wide range of plate kinematic conditions. We need to better understand what the drivers behind rift kinematics are and how these kinematics affect the evolution of rift systems (e.g. Brune et al., 2016).
-
Mantle. Much research has focused on the crust and lithosphere, which are easier to access and study, but the influence of the mantle on rifting remains elusive although tectonic modelling suggests it can have a dominant impact (e.g. Chenin et al., 2015; Zwaan et al., 2022), and more research is greatly needed.
To approach these research topics, existing and novel methods need to be applied. Fieldwork in rift systems remains a key means of acquiring data, but its effectiveness can be considerably enhanced by the use of drones and detailed satellite imagery. Such satellite imagery (e.g. topography data), for instance, allows automatic interpretation using state-of-the-art algorithms and artificial intelligence (AI) to generate fault maps (Gayrin et al., 2023). Machine learning and AI in general will expand our research options in many different ways (Chen et al., 2023). Even so, such advanced methods will rely on detailed and correct data from the natural world, which remains a challenge. For instance, satellite imagery allows bathymetry mapping of lakes and seas, which cover large parts of the world's rift systems, yet high-resolution bathymetric mapping of lake floors or seafloors can only be obtained via sonar surveys during scientific cruises (Wölfl et al., 2019). Such cruises will also remain crucial for sampling the deep through seafloor dragging or the acquisition of seismic datasets.
Offshore and onshore drilling of the lithosphere in rift settings, as done by the International Ocean Discovery Program (IODP) and International Continental Scientific Drilling Program (ICDP), respectively, continue to draw new challenges and targets (Koppers and Coggon, 2020), providing new insights and research opportunities (e.g. the proposed ICDP ADD-ON to drill into the Afar Rift; ADD-ON, 2023). Similarly, large-scale geophysical efforts can provide the detailed data that allow us to better understand the subsurface geology in rift systems. A wealth of otherwise undisclosed geological information such as borehole logs and seismic surveys can also be obtained from industry partners such as energy companies, mining operators, and water production firms (Peron-Pinvidic et al., 2019). Indeed, increasing collaboration and exchange of information and ideas between industry and academia, as well as interaction with policymakers, may be the way to move science forward (Ankrah and AL-Tabbaa, 2015; Ludden, 2020) (see also Sects. 5.2 and 5.3).
Next to advancing data acquisition methods and increased collaboration, geological modelling approaches, either in the laboratory (analogue) or using numerical codes, provide a unique means to better appreciate the long-term evolution of rift systems and the associated geological processes. Both approaches are rapidly developing, and, in the ideal case, researchers can combine them to get “the best of both worlds” (e.g. Brune et al., 2017; Maestrelli et al., 2022). Such interdisciplinary research, also beyond the modelling domain, poses great opportunities for advancing our knowledge of rift systems.
Another exciting research direction is the developing research field that aims at deformation on planetary bodies. Satellite imagery and geophysical analyses done by orbital and landing probes allow us to study the planets and moons in our solar system, which in various cases contain rift-like structures. Researchers have, for instance, identified rifts on Mars (Hauber et al., 2010), Venus (Regorda et al., 2023), and Mercury (Watters et al., 2016). Studying such planetary rift tectonics may also provide insights into early terrestrial tectonics that operated under very different conditions than in the present day (Bradley, 2008; Capitanio et al., 2019).
5.2 Natural hazards
Dealing with the risks posed by natural hazards in rift systems (seismicity, volcanism, and mass wasting; see Sect. 3) requires a thorough understanding of the geological processes causing these hazards (see Sects. 2 and 5.1). However, in order to understand a specific hazard and the risk that it poses in a specific area, researchers and the government can profit from much more detailed study and especially monitoring approaches. In the case of those geo-hazards related to earthquakes, volcanism, and mass wasting, detailed monitoring takes place through a combination of field observations, geophysical methods (earthquake monitoring and analysis through permanent seismic networks and seismic data interpretation), satellite imagery analysis (incl. InSAR), and stress measurements in boreholes (see Sects. 3.1.2 and 3.2.2). For estimating landslide risks, the impact of human activity (agriculture and deforestation) also needs to be assessed (e.g. Depicker et al., 2021).
Installing and expanding monitoring networks in known risk areas around the globe is clearly of great societal interest, but another important challenge for the future may be the assessment of hazards in less obvious locations. This is of particular relevance when it comes to intra-plate earthquakes along “passive” rifted margins or in old and (supposedly) tectonically inactive rift basins or when earthquake cycles or volcanism with very long recurrence times are involved (see Sect. 3.1). Here, multidisciplinary approaches can help to provide the best possible risk assessment. A promising way to expand our risk assessment capacities, in particular in poorer regions in the world, is by setting up innovative monitoring networks that involve active participation of the local community (citizen science; e.g. Boudoire et al., 2022; Sekajugo et al., 2022). This is especially relevant to populations living in the East African Rift System which are projected to rise considerably for the foreseeable future (Worldometer, 2023). Furthermore, although there are distinct limitations (Mancini et al., 2022), there are great opportunities to streamline analysis and improve risk pattern recognition through machine learning algorithms and AI that can recognise patterns in earthquake catalogues (e.g. Stockman et al., 2023; Zlydenko et al., 2023).
Another key means to test risks posed by geological processes in rift systems is to perform detailed modelling of these processes and link them to observations from nature. For example, Corbi et al. (2019) show how machine learning can predict earthquakes in analogue models, which could potentially be used for real earthquake forecasting. Likewise, modellers can use a variety of methods to simulate volcanic processes (Poppe et al., 2022), (submarine) landslides, and associated tsunamis (e.g. Berndt et al., 2009; McFall and Fritz, 2016). The output of such interdisciplinary analyses can serve to improve existing hazard and risk assessments. This is especially relevant for human activities that involve subsurface fluid injection, such as geothermal energy projects and unconventional hydrocarbon production, which regularly generate seismicity (e.g. Andrés et al., 2019).
5.3 Geo-resources
The energy transition will require huge amounts of geo-resources, which poses significant challenges and opportunities for research and development. Exploration and production of such resources will have to be stepped up by an order of magnitude, and new areas need to be explored to satisfy the demand posed by a growing global population that is increasing its overall level of development (e.g. IEA, 2021; UNEP, 2023). For instance, the European Union has expressed its intention to increase the extraction of mineral resources from within the European continent (EU, 2023), and the USA has expressed similar intentions (USDC, 2019).
A crucial challenge for geoscientists within this context will be to improve our understanding of the processes leading to the generation of mineral deposits in rift systems and to develop new methods to trace down and exploit these mineral resources as efficiently and minimally invasively as possible. Many of the best-accessible deposits found near the Earth's surface have long since been discovered, but a wealth of mineral deposits is expected to be found deeper in the subsurface (Arndt et al., 2017). Though technically challenging, deeper mining activities have the advantage of diminishing the impact on the landscape and environment, making such activities more sustainable (Arndt et al., 2017). A promising avenue for mineral exploration is the “mineral system analysis” approach, somewhat similar to the analysis of petroleum systems (Sect. 4.2.1), where the full context of mineral deposit development is being assessed, thus allowing a better understanding of where mineral exploration should focus (e.g. Hagemann et al., 2016; Lawley et al., 2022). Furthermore, ongoing exploration of the offshore parts of rift systems will provide a whole new mining environment, although such mining is still highly challenging and may have major consequences for crucial deep-sea ecosystems (Washburn et al., 2019; Kung et al., 2021). As such, there are also great opportunities here for the development of more sustainable mining or extraction methods.
Energy transition policies also imply a definitive move away from fossil fuels, yet hydrocarbons will remain an important part of the global energy mix for the foreseeable future (Kober et al., 2020; IEA, 2023b). Hydrocarbon exploration is likely to continue in many places (e.g. in the failed rifts crossing the African continent), but, in the future, energy systems that combine the use of renewable energy and CCS techniques can render the production of hydrocarbons more efficient, sustainable, and potentially even carbon neutral (IEA, 2023a). Offshore hydrocarbon production in rift environments could similarly become sustainable, especially when targeting the production of natural gas (potentially in hydrate form; see Sect. 4.2.1) instead of the more polluting petroleum and coal.
Natural H2 is a true wildcard in the energy transition. Still very much underexplored, the vast expanses of exhumed mantle rocks at rifted margins imply that huge volumes of natural H2 are formed during rifting (Liu et al., 2023). Perhaps, as Gaucher (2020) argues, the coming years will see the start of a flourishing natural H2 industry. However, in order to successfully develop such an industry, upcoming natural H2 exploration efforts should aim at identifying the crucial aspects of potential H2 systems, which are rather similar in nature to those in petroleum systems (Sect. 4.2.2; Gaucher et al., 2023; Zwaan et al., 2023). This fact presents great opportunities, since very similar exploration methods to the ones used in the petroleum industry can be applied for the development of this sustainable energy source.
Geothermal energy is an ever-developing and sustainable geo-energy source with great potential, as already demonstrated in, for instance, Iceland (Sect. 4.2.3). However, the huge geothermal potential in the largest magma-rich continental rift system in the world, the East African Rift System (e.g. Elbarbary et al., 2022), is gathering interest but remains mostly untapped (e.g. IRENA, 2020). Future efforts to unlock these energy resources will involve the detailed assessment of the geothermal regime and subsurface geology in the various rift basins in eastern Africa, with particular attention to the highly magmatic Afar Rift, where very little data are available but high heat flows are recorded (Limberger et al., 2018; IHFC, 2023). There may even be possibilities of setting up local geothermal projects that can support the development of local communities (Varet, 2018). Moreover, systematical extraction of dissolved minerals and elements from geothermal fluids provides a means to add value to geothermal operations (Kölbel et al., 2023). Geothermal plants in Iceland have also been shown to emit large volumes of natural H2 during their operations, which could be captured and used as an additional green energy source (Gaucher et al., 2023). Similar natural H2 emissions have been recorded in Afar, as well, providing an additional motivation to explore resources in that specific region (Pasquet et al., 2021).
Future energy requirements will demand a large increase in temporary storage capacity (Duffy et al., 2023), and attempts to reduce the concentration of atmospheric CO2, which is currently still steeply rising, will require vast expansion of permanent sequestration capacity (Tucker, 2018). Our best hope of realising these requirements is to identify the most promising sites for either type of storage and to expand the storage capacity of these sites as much as possible. A caveat is that both types of storage will likely have to be close to industrial centres, where resources are consumed and CO2 is produced. A solution may be to focus temporary storage efforts on evaporite deposits and depleted hydrocarbon reservoirs, many of which are found in rift settings, whereas CCS could focus on the widely available exhumed mantle bodies and basaltic flows around the globe (Matter and Kelemen, 2009).
Upcoming research into water resources may target subsurface water flows from rift shoulders into rift basins and offshore aquifers along rifted margins (Micallef et al., 2021). Knowledge of these water systems will be critical to fulfil the water needs of the ever-growing populations living in rift environments and may also be important for developing local geothermal projects (Varet, 2018). Another important research topic will be the impact of these growing populations, and the impact of the associated intensified land use, on soils in terms of their capacity to yield sufficiently large harvests to sustain these populations. More intense land use, deforestation, and soil degradation will increase the risks posed by natural hazards such as landslides (e.g. Depicker et al., 2021).
Finally, we believe that a crucial aspect of successful future geo-resource endeavours is the efficient exchange of knowledge and expertise between researchers and industry players, as well as government agencies. Such knowledge transfer benefits all involved, as it allows researchers to better understand the geo-resource at hand, be it minerals, hydrocarbons, or natural hydrogen, which companies can use to improve their exploration strategies, and governments can profit from the resulting economic activity. Excellent examples are the DINOloket and NLog platforms that collect and make available data regarding the subsurface of the Netherlands (TNO, 2024a, b). The DISKOS and NPD Factpages platforms are similar efforts to make available data regarding the Norwegian subsurface (NPD, 2024a, b), with NOPIMS being another example from Australia (Geoscience Australia, 2024).
Rifting and continental break-up form a key research topic within geosciences. A thorough understanding of the processes involved and of the associated natural hazards and natural resources is of great importance to both science and society. In this review we provided an up-to-date summary of these processes, hazards, and resources. In addition to reviewing the state of the art in rift research, we also discussed the key challenges for the future and identified opportunities for research and knowledge application, where especially knowledge transfer between science, industry, and government can help realise breakthroughs. We therefore hope that this review paper will inspire future research in the field of rifting.
The data used for several of the figures included in this paper can be obtained from the publications and repositories cited in the relevant figure captions.
Conceptualisation: all authors. Project administration: FZ. Visualisation: PC, JJJP, and SB. Writing (original draft preparation; review and editing): all authors.
The contact author has declared that none of the authors has any competing interests.
Publisher's note: Copernicus Publications remains neutral with regard to jurisdictional claims made in the text, published maps, institutional affiliations, or any other geographical representation in this paper. While Copernicus Publications makes every effort to include appropriate place names, the final responsibility lies with the authors.
This article is part of the special issue “(D)rifting into the future: the relevance of rifts and divergent margins in the 21st century”. It is not associated with a conference.
We acknowledge support from the GFZ Discovery Fund, Petrobras, the European Union, the Helmholtz Recruitment Initiative programme, the Helmholtz Centre Potsdam – GFZ German Research Centre for Geosciences, the Ministry of Science and Innovation of Spain, and Cardiff University. Moreover, we would like to thank editor Yang Chu and two anonymous reviewers for their helpful feedback.
Frank Zwaan was funded by a GFZ Discovery Fund fellowship. Tiago M. Alves benefited from funding from the Petrobras “Rift in Sub-salt Successions” grant (project no. 523109) awarded to Cardiff University. Patricia Cadenas has received funding from the European Union’s Horizon 2020 research and innovation programme under Marie Skłodowska-Curie grant agreement no. 895895, project SUBIMAP. Anne Glerum received funding from a Helmholtz Recruitment Initiative. Sascha Brune was funded by the European Union (ERC, EMERGE, 101087245).
The article processing charges for this open-access publication were split between (1) the Helmholtz Centre Potsdam – GFZ German Research Centre for Geosciences, (2) research project ASTRACAN (ref. MCIU-22-PID2021-123116NB-I00) funded by the Ministry of Science and Innovation of Spain, and (3) the Cardiff University Institutional Open Access Fund (ref. 2024-OA-0498).
This paper was edited by Yang Chu and reviewed by two anonymous referees.
ADD-ON – Afar Dallol Drilling – ONset of sedimentary processes in an ac4ve rif basin: https://www.afardalloldrilling.com (last access: 11 July 2024), 2023. a
Agartan, E., Gaddipati, M., Yip, Y., Savage, B., and Ozgen, C.: CO2 storage in depleted oil and gas fields in the Gulf of Mexico, Int. J. Greenh. Gas Con., 72, 38–48, https://doi.org/10.1016/j.ijggc.2018.02.022, 2018. a
Agostini, A., Bonini, M., Corti, G., Sani, F., and Mazzarini, F.: Fault architecture in the Main Ethiopian Rift and comparison with experimental models: Implications for rift evolution and Nubia–Somalia kinematics, Earth Planet. Sc. Lett., 301, 479–492, https://doi.org/10.1016/j.epsl.2010.11.024, 2011. a, b
Ahmed, A. H. and Arai, S.: Platinum-group Minerals in Podiform Chromitites of the Oman Ophiolite, Can. Mineral., 41, 597–616, https://doi.org/10.2113/gscanmin.41.3.597, 2003. a
Ajanovic, A., Sayer, M., and Haas, R.: The economics and the environmental benignity of different colors of hydrogen, Int. J. Hydrogen Energ., 47, 24136–24154, https://doi.org/10.1016/j.ijhydene.2022.02.094, 2022. a
Ajayi, T., Gomes, J. S., and Bera, A.: A review of CO2 storage in geological formations emphasizing modeling, monitoring and capacity estimation approaches, Petrol. Sci., 16, 1028–1063, https://doi.org/10.1007/s12182-019-0340-8, 2019. a, b
Akçar, N., Tikhomirov, D., Özkaymak, Ç., Ivy-Ochs, S., Alfimov, V., Sözbilir, H., Uzel, B., and Schlüchter, C.: 36Cl exposure dating of paleoearthquakes in the Eastern Mediterranean: First results from the western Anatolian Extensional Province, Manisa fault zone, Turkey, GSA Bulletin, 124, 1724–1735, https://doi.org/10.1130/B30614.1, 2012. a, b
Albers, E., Bach, W., Pérez-Gussinyé, M., McCammon, C., and Frederichs, T.: Serpentinization-driven H2 production from continental break-up to mid-Ocean Ridge spreading: Unexpected high rates at the West Iberia margin, Front. Earth Sci., 9, 673063, https://doi.org/10.3389/feart.2021.673063, 2021. a, b, c
Alewell, C., Ringeval, B., Ballabio, C., Robinson, D. A., Panagos, P., and Borrelli, P.: Global phosphorus shortage will be aggravated by soil erosion, Nat. Commun., 11, 4546, https://doi.org/10.1038/s41467-020-18326-7, 2020. a
Alves, T., Fetter, M., Busby, C., Gontijo, R., Cunha, T. A., and Mattos, N. H.: A tectono-stratigraphic review of continental breakup on intraplate continental margins and its impact on resultant hydrocarbon systems, Mar. Petrol. Geol., 117, 104341, https://doi.org/10.1016/j.marpetgeo.2020.104341, 2020. a, b, c
Ambraseys, N. N.: Earthquakes and archaeology, J. Archaeol. Sci., 33, 1008–1016, https://doi.org/10.1016/j.jas.2005.11.006, 2006. a
Ambraseys, N. N. and Adams, R. D.: Reappraisal of major African earthquakes, south of 20° N, 1900–1930, Nat. Hazards, 4, 389–419, https://doi.org/10.1007/BF00126646, 1991. a, b, c, d
Andrés, S., Santillán, D., Mosquera, J. C., and Cueto-Felgueroso, L.: Thermo-Poroelastic Analysis of Induced Seismicity at the Basel Enhanced Geothermal System, Sustainability: Science Practice and Policy, 11, 6904, https://doi.org/10.3390/su11246904, 2019. a
Andrés-Martínez, M., Pérez-Gussinyé, M., Armitage, J., and Morgan, J. P.: Thermomechanical Implications of Sediment Transport for the Architecture and Evolution of Continental Rifts and Margins, Tectonics, 38, 641–665, https://doi.org/10.1029/2018TC005346, 2019. a
Ankrah, S. and AL-Tabbaa, O.: Universities–industry collaboration: A systematic review, Scand. J. Manag., 31, 387–408, https://doi.org/10.1016/j.scaman.2015.02.003, 2015. a
Arndt, N. T., Fontboté, L., Hedenquist, J. W., Kesler, S. E., Thompson, J. F. H., and Wood, D. G.: Future Global Mineral Resources, Geochem. Perspect., 6, 1–171, https://doi.org/10.7185/geochempersp.6.1, 2017. a, b
ArRajehi, A., McClusky, S., Reilinger, R., Daoud, M., Alchalbi, A., Ergintav, S., Gomez, F., Sholan, J., Bou-Rabee, F., Ogubazghi, G., Haileab, B., Fisseha, S., Asfaw, L., Mahmoud, S., Rayan, A., Bendik, R., and Kogan, L.: Geodetic constraints on present-day motion of the Arabian Plate: Implications for Red Sea and Gulf of Aden rifting, Tectonics, 29, TC3011, https://doi.org/10.1029/2009tc002482, 2010. a, b
Arzhannikova, A. V., Arzhannikov, S. G., Ritz, J.-F., Chebotarev, A. A., and Yakhnenko, A. S.: Earthquake geology of the Mondy fault (SW Baikal Rift, Siberia), J. Asian Earth Sci., 248, 105614, https://doi.org/10.1016/j.jseaes.2023.105614, 2023. a
Assier-Rzadkieaicz, S., Heinrich, P., Sabatier, P. C., Savoye, B., and Bourillet, J. F.: Numerical Modelling of a Landslide-generated Tsunami: The 1979 Nice Event, Pure Appl. Geophys., 157, 1707–1727, https://doi.org/10.1007/PL00001057, 2000. a
Augustin, N., Devey, C. W., van der Zwan, F. M., Feldens, P., Tominaga, M., Bantan, R. A., and Kwasnitschka, T.: The rifting to spreading transition in the Red Sea, Earth Planet. Sc. Lett., 395, 217–230, https://doi.org/10.1016/j.epsl.2014.03.047, 2014. a
Aydan, Ö. and Kumsar, H.: Assessment of the earthquake potential of the west Aegean region of Turkey based on seismicity, tectonics, crustal deformation and geo-archaeological evidence and its geotechnical aspects, B. Eng. Geol. Environ., 74, 1037–1055, https://doi.org/10.1007/s10064-014-0684-7, 2015. a
Aydin, H. and Merey, S.: Potential of geothermal energy production from depleted gas fields: A case study of Dodan Field, Turkey, Renew. Energ., 164, 1076–1088, https://doi.org/10.1016/j.renene.2020.10.057, 2021. a
Bajpai, S., Shreyash, N., Singh, S., Memon, A. R., Sonker, M., Tiwary, S. K., and Biswas, S.: Opportunities, challenges and the way ahead for carbon capture, utilization and sequestration (CCUS) by the hydrocarbon industry: Towards a sustainable future, Energy Reports, 8, 15595–15616, https://doi.org/10.1016/j.egyr.2022.11.023, 2022. a, b, c
Barnard, L.: Lava barriers partially protect Icelandic town, Construction Briefing, https://www.constructionbriefing.com/news/lava-barriers-partially-protect-icelandic-town/8034345.article (last access: 11 July 2024), 2024. a
Barrie, C. T. and Hannington, M. D.: Classification of Volcanic-Associated Massive Sulfide Deposits Based on Host-Rock Composition, in: Volcanic Associated Massive Sulfide Deposits: Processes and Examples in Modern and Ancient Settings, vol. 8, Society of Economic Geologists, https://doi.org/10.5382/Rev.08.01, 1997. a
Bažant, Z. P., Salviato, M., Chau, V. T., Viswanathan, H., and Zubelewicz, A.: Why Fracking Works, J. Appl. Mech., 81, 101010, https://doi.org/10.1115/1.4028192, 2014. a
Bekele, A. and Schmerold, R.: Characterization of brines and evaporite deposits for their lithium contents in the northern part of the Danakil Depression and in some selected areas of the Main Ethiopian Rift lakes, J. Afr. Earth Sci., 170, 103904, https://doi.org/10.1016/j.jafrearsci.2020.103904, 2020. a
Benabdellouahed, M., Klingelhoefer, F., Gutscher, M.-A., Rabineau, M., Biari, Y., Hafid, M., Duarte, J. C., Schnabel, M., Baltzer, A., Pedoja, K., Le Roy, P., Reichert, C., and Sahabi, M.: Recent uplift of the Atlantic Atlas (offshore West Morocco): Tectonic arch and submarine terraces, Tectonophysics, 706–707, 46–58, https://doi.org/10.1016/j.tecto.2017.03.024, 2017. a
Benes, V. and Scott, S. D.: Oblique rifting in the Havre Trough and its propagation into the continental margin of New Zealand: Comparison with analogue experiments, Mar. Geophys. Res., 18, 189–201, https://doi.org/10.1007/BF00286077, 1996. a
Berndt, C., Brune, S., Nisbet, E., Zschau, J., and Sobolev, S. V.: Tsunami modeling of a submarine landslide in the Fram Strait, Geochem. Geophy. Geosy., 10, Q04009, https://doi.org/10.1029/2008gc002292, 2009. a
Berndt, C., Planke, S., Teagle, D., Huismans, R., Torsvik, T., Frieling, J., Jones, M. T., Jerram, D. A., Tegner, C., Faleide, J. I., Coxall, H., and Hong, W.-L.: Northeast Atlantic breakup volcanism and consequences for Paleogene climate change – MagellanPlus Workshop report, Sci. Dril., 26, 69–85, https://doi.org/10.5194/sd-26-69-2019, 2019. a
Bialas, R. W. and Buck, W. R.: How sediment promotes narrow rifting: Application to the Gulf of California, Tectonics, 28, TC4014, https://doi.org/10.1029/2008TC002394, 2009. a
Biggs, J., Ayele, A., Fischer, T. P., Fontijn, K., Hutchison, W., Kazimoto, E., Whaler, K., and Wright, T. J.: Volcanic activity and hazard in the East African Rift Zone, Nat. Commun., 12, 6881, https://doi.org/10.1038/s41467-021-27166-y, 2021. a, b, c
Bird, P.: An updated digital model of plate boundaries, Geochem. Geophy. Geosy., 4, 1027, https://doi.org/10.1029/2001GC000252, 2003. a
Blowes, D. W., Ptacek, C. J., Jambor, J. L., Weisener, C. G., Paktunc, D., Gould, W. D., and Johnson, D. B.: 11.5 – The Geochemistry of Acid Mine Drainage, in: Treatise on Geochemistry, 2nd edn., edited by: Holland, H. D. and Turekian, K. K., Elsevier, Oxford, 131–190, https://doi.org/10.1016/B978-0-08-095975-7.00905-0, 2014. a
Bochneva, A., Lalomov, A., and LeBarge, W.: Placer mineral deposits of Russian Arctic zone: Genetic prerequisites of formation and prospect of development of mineral resources, Ore Geol. Rev., 138, 104349, https://doi.org/10.1016/j.oregeorev.2021.104349, 2021. a
Bondevik, S., Løvholt, F., Harbitz, C., Mangerud, J., Dawson, A., and Svendsen, J. I.: The Storegga Slide tsunami – comparing field observations with numerical simulations, Mar. Petrol. Geol., 22, 195–208, https://doi.org/10.1016/j.marpetgeo.2004.10.003, 2005. a
Bonini, L., Fracassi, U., Bertone, N., Maesano, F. E., Valensise, G., and Basili, R.: How do inherited dip-slip faults affect the development of new extensional faults? Insights from wet clay analog models, J. Struct. Geol., 169, 104836, https://doi.org/10.1016/j.jsg.2023.104836, 2023. a
Boudoire, G., Calabrese, S., Colacicco, A., Sordini, P., Habakaramo Macumu, P., Rafflin, V., Valade, S., Mweze, T., Kazadi Mwepu, J.-C., Safari Habari, F., Amani Kahamire, T., Mumbere Mutima, Y., Ngaruye, J.-C., Tuyishime, A., Tumaini Sadiki, A., Mavonga Tuluka, G., Mapendano Yalire, M., Kets, E.-D., Grassa, F., D'Alessandro, W., Caliro, S., Rufino, F., and Tedesco, D.: Scientific response to the 2021 eruption of Nyiragongo based on the implementation of a participatory monitoring system, Sci. Rep.-UK, 12, 7488, https://doi.org/10.1038/s41598-022-11149-0, 2022. a
Boudoire, G., Pasdeloup, G., Schiavi, F., Cluzel, N., Rafflin, V., Grassa, F., Giuffrida, G., Liuzzo, M., Harris, A., Laporte, D., and Rizzo, A. L.: Magma storage and degassing beneath the youngest volcanoes of the Massif Central (France): Lessons for the monitoring of a dormant volcanic province, Chem. Geol., 634, 121603, https://doi.org/10.1016/j.chemgeo.2023.121603, 2023. a, b
Bourassa, A. E., Robock, A., Randel, W. J., Deshler, T., Rieger, L. A., Lloyd, N. D., Llewellyn, E. J. T., and Degenstein, D. A.: Large volcanic aerosol load in the stratosphere linked to Asian monsoon transport, Science, 337, 78–81, https://doi.org/10.1126/science.1219371, 2012. a
Bradley, D. C.: Passive margins through earth history, Earth-Sci. Rev., 91, 1–26, https://doi.org/10.1016/j.earscirev.2008.08.001, 2008. a, b
Broeckx, J., Vanmaercke, M., Duchateau, R., and Poesen, J.: A data-based landslide susceptibility map of Africa, Earth-Sci. Rev., 185, 102–121, https://doi.org/10.1016/j.earscirev.2018.05.002, 2018. a
Brown, A. C.: 13.10 – Low-Temperature Sediment-Hosted Copper Deposits, in: Treatise on Geochemistry, 2nd edn., edited by: Holland, H. D. and Turekian, K. K., Elsevier, Oxford, 251–271, https://doi.org/10.1016/B978-0-08-095975-7.01110-4, 2014. a
Brun, J. P.: Narrow rifts versus wide rifts: inferences for the mechanics of rifting from laboratory experiments, Philos. T. Roy. Soc. A, 357, 695–712, https://doi.org/10.1098/rsta.1999.0349, 1999. a, b, c
Brun, J.-P., Sokoutis, D., Tirel, C., Gueydan, F., Van Den Driessche, J., and Beslier, M.-O.: Crustal versus mantle core complexes, Tectonophysics, 746, 22–45, https://doi.org/10.1016/j.tecto.2017.09.017, 2018. a, b, c, d
Brune, S.: Rifts and Rifted Margins, in: Plate Boundaries and Natural Hazards, Geophysical Monograph Series, American Geophysical Union, 11–37, https://doi.org/10.1002/9781119054146.ch2, 2016. a, b, c
Brune, S., Williams, S. E., Butterworth, N. P., and Müller, R. D.: Abrupt plate accelerations shape rifted continental margins, Nature, 536, 201–204, https://doi.org/10.1038/nature18319, 2016. a, b
Brune, S., Corti, G., and Ranalli, G.: Controls of inherited lithospheric heterogeneity on rift linkage: Numerical and analog models of interaction between the Kenyan and Ethiopian rifts across the Turkana depression, Tectonics, 36, 1767–1786, https://doi.org/10.1002/2017TC004739, 2017. a
Brune, S., Kolawole, F., Olive, J.-A., Stamps, D. S., Buck, W. R., Buiter, S. J. H., Furman, T., and Shillington, D. J.: Geodynamics of continental rift initiation and evolution, Nature Reviews Earth & Environment, 4, 235–253, https://doi.org/10.1038/s43017-023-00391-3, 2023. a
Buck, W. R.: Modes of continental lithospheric extension, J. Geophys. Res.-Sol. Ea., 96, 20161–20178, https://doi.org/10.1029/91JB01485, 1991. a, b, c
Buck, W. R.: The role of magma in the development of the Afro-Arabian Rift System, Geological Society, London, Special Publications, 259, 43–54, https://doi.org/10.1144/GSL.SP.2006.259.01.05, 2006. a, b
Buck, W. R.: The role of magmatic loads and rift jumps in generating seaward dipping reflectors on volcanic rifted margins, Earth Planet. Sc. Lett., 466, 62–69, https://doi.org/10.1016/j.epsl.2017.02.041, 2017. a, b
Buck, W. R., Lavier, L. L., and Poliakov, A. N. B.: Modes of faulting at mid-ocean ridges, Nature, 434, 719–723, https://doi.org/10.1038/nature03358, 2005. a
Buiter, S. J. H. and Torsvik, T. H.: A review of Wilson Cycle plate margins: A role for mantle plumes in continental break-up along sutures?, Gondwana Res., 26, 627–653, https://doi.org/10.1016/j.gr.2014.02.007, 2014. a
Buiter, S. J. H., Brune, S., Keir, D., and Peron-Pinvidic, G.: Chapter 19 – Rifting Continents, in: Dynamics of Plate Tectonics and Mantle Convection, edited by: Duarte, J. C., Elsevier, 459–481, https://doi.org/10.1016/B978-0-323-85733-8.00016-0, 2023. a
Bungum, H., Olesen, O., Pascal, C., Gibbons, S., Lindholm, C., and VestØl, O.: To what extent is the present seismicity of Norway driven by post-glacial rebound?, J. Geol. Soc. London, 167, 373–384, https://doi.org/10.1144/0016-76492009-009, 2010. a
Burisch, M., Markl, G., and Gutzmer, J.: Breakup with benefits – hydrothermal mineral systems related to the disintegration of a supercontinent, Earth Planet. Sc. Lett., 580, 117373, https://doi.org/10.1016/j.epsl.2022.117373, 2022. a
Burov, E. and Cloetingh, S.: Erosion and rift dynamics: new thermomechanical aspects of post-rift evolution of extensional basins, Earth Planet. Sc. Lett., 150, 7–26, https://doi.org/10.1016/S0012-821X(97)00069-1, 1997. a
Burov, E. B. and Watts, A. B.: The long-term strength of continental lithosphere:“jelly sandwich” or “crème brûlée”?, GSA Today, 16, 4–10, https://doi.org/10.1130/1052-5173(2006)016<4:TLTSOC>2.0.CO;2, 2006. a
Calais, E., Freed, A. M., Van Arsdale, R., and Stein, S.: Triggering of New Madrid seismicity by late-Pleistocene erosion, Nature, 466, 608–611, https://doi.org/10.1038/nature09258, 2010. a
Camelbeeck, T. and Eck, T.: The Roer Valley Graben earthquake of 13 April 1992 and its seismotectonic setting, Terra Nova, 6, 291–300, https://doi.org/10.1111/j.1365-3121.1994.tb00499.x, 1994. a
Cannat, M., Sauter, D., Mendel, V., Ruellan, E., Okino, K., Escartin, J., Combier, V., and Baala, M.: Modes of seafloor generation at a melt-poor ultraslow-spreading ridge, Geology, 34, 605–608, https://doi.org/10.1130/G22486.1, 2006. a
Cantrell, L. and Young, M.: Fatal fall into a volcanic fumarole, Wilderness Environ. Med., 20, 77–79, https://doi.org/10.1580/08-WEME-CR-199.1, 2009. a
Capitanio, F. A., Nebel, O., Cawood, P. A., Weinberg, R. F., and Chowdhury, P.: Reconciling thermal regimes and tectonics of the early Earth, Geology, 47, 923–927, https://doi.org/10.1130/G46239.1, 2019. a
Castro, R. R., Carciumaru, D. D., Collin, M., Vetel, W., Gonzalez-Huizar, H., Mendoza, A., and Pérez-Vertti, A.: Seismicity in the Gulf of California, Mexico, in the period 1901–2018, J. S. Am. Earth Sci., 106, 103087, https://doi.org/10.1016/j.jsames.2020.103087, 2021. a
Catuneanu, O., Abreu, V., Bhattacharya, J. P., Blum, M. D., Dalrymple, R. W., Eriksson, P. G., Fielding, C. R., Fisher, W. L., Galloway, W. E., Gibling, M. R., Giles, K. A., Holbrook, J. M., Jordan, R., Kendall, C. G. S. C., Macurda, B., Martinsen, O. J., Miall, A. D., Neal, J. E., Nummedal, D., Pomar, L., Posamentier, H. W., Pratt, B. R., Sarg, J. F., Shanley, K. W., Steel, R. J., Strasser, A., Tucker, M. E., and Winker, C.: Towards the standardization of sequence stratigraphy, Earth-Sci. Rev., 92, 1–33, https://doi.org/10.1016/j.earscirev.2008.10.003, 2009. a
Chen, G., Cheng, Q., and Puetz, S.: Special Issue: Data-Driven Discovery in Geosciences: Opportunities and Challenges, Math. Geosci., 55, 287–293, https://doi.org/10.1007/s11004-023-10054-0, 2023. a
Chen, X., Nakata, N., Pennington, C., Haffener, J., Chang, J. C., He, X., Zhan, Z., Ni, S., and Walter, J. I.: The Pawnee earthquake as a result of the interplay among injection, faults and foreshocks, Sci. Rep.-UK, 7, 4945, https://doi.org/10.1038/s41598-017-04992-z, 2017. a
Chenin, P., Manatschal, G., Lavier, L. L., and Erratt, D.: Assessing the impact of orogenic inheritance on the architecture, timing and magmatic budget of the North Atlantic rift system: a mapping approach, J. Geol. Soc. London, 172, 711–720, https://doi.org/10.1144/jgs2014-139, 2015. a, b
Chenin, P., Picazo, S., Jammes, S., Manatschal, G., Müntener, O., and Karner, G.: Potential role of lithospheric mantle composition in the Wilson cycle: a North Atlantic perspective, Geological Society, London, Special Publications, 470, 157–172, https://doi.org/10.1144/SP470.10, 2019. a
Cherkose, B. A., Issa, S., Saibi, H., and ElHaj, K.: Building a geospatial model to identify potential geothermal sites in Ayrobera: Afar depression, NE Ethiopia, Geothermics, 110, 102689, https://doi.org/10.1016/j.geothermics.2023.102689, 2023. a
Clark, S. H. B., Poole, F. G., and Wang, Z.: Comparison of some sediment-hosted, stratiform barite deposits in China, the United States, and India, Ore Geol. Rev., 24, 85–101, https://doi.org/10.1016/j.oregeorev.2003.08.009, 2004. a
Clarke, B., Uken, R., and Reinhardt, J.: Structural and compositional constraints on the emplacement of the Bushveld Complex, South Africa, Lithos, 111, 21–36, https://doi.org/10.1016/j.lithos.2008.11.006, 2009. a
Clift, P. D., Brune, S., and Quinteros, J.: Climate changes control offshore crustal structure at South China Sea continental margin, Earth Planet. Sc. Lett., 420, 66–72, https://doi.org/10.1016/j.epsl.2015.03.032, 2015. a
Cooper, C. L., Savov, I. P., Patton, H., Hubbard, A., Ivanovic, R. F., Carrivick, J. L., and Swindles, G. T.: Is there a climatic control on Icelandic volcanism?, Quaternary Science Advances, 1, 100004, https://doi.org/10.1016/j.qsa.2020.100004, 2020. a
Corbi, F., Sandri, L., Bedford, J., Funiciello, F., Brizzi, S., Rosenau, M., and Lallemand, S.: Machine learning can predict the timing and size of analog earthquakes, Geophys. Res. Lett., 46, 1303–1311, https://doi.org/10.1029/2018gl081251, 2019. a
Corti, G.: Evolution and characteristics of continental rifting: Analog modeling-inspired view and comparison with examples from the East African Rift System, Tectonophysics, 522–523, 1–33, https://doi.org/10.1016/j.tecto.2011.06.010, 2012. a, b
Corti, G., Bonini, M., Sokoutis, D., Innocenti, F., Manetti, P., Cloetingh, S., and Mulugeta, G.: Continental rift architecture and patterns of magma migration: A dynamic analysis based on centrifuge models, Tectonics, 23, TC2012, https://doi.org/10.1029/2003tc001561, 2004. a
Corti, G., van Wijk, J., Cloetingh, S., and Morley, C. K.: Tectonic inheritance and continental rift architecture: Numerical and analogue models of the East African Rift system, Tectonics, 26, TC6006, https://doi.org/10.1029/2006tc002086, 2007. a
Craig, T. J., Jackson, J. A., Priestley, K., and McKenzie, D.: Earthquake distribution patterns in Africa: their relationship to variations in lithospheric and geological structure, and their rheological implications, Geophys. J. Int., 185, 403–434, https://doi.org/10.1111/j.1365-246X.2011.04950.x, 2011. a
Dahlkamp, F. J.: Uranium deposits of the world, Springer Berlin Heidelberg, Berlin, Heidelberg, https://doi.org/10.1007/978-3-540-78558-3, 2009. a, b
Dahm, T., Stiller, M., Mechie, J., Heimann, S., Hensch, M., Woith, H., Schmidt, B., Gabriel, G., and Weber, M.: Seismological and Geophysical Signatures of the Deep Crustal Magma Systems of the Cenozoic Volcanic Fields Beneath the Eifel, Germany, Geochem. Geophy. Geosy., 21, e2020GC009062, https://doi.org/10.1029/2020GC009062, 2020. a
Danabalan, D., Gluyas, J. G., Macpherson, C. G., Abraham-James, T. H., Bluett, J. J., Barry, P. H., and Ballentine, C. J.: The principles of helium exploration, Petrol. Geosci., 28, etgeo2021-029, https://doi.org/10.1144/petgeo2021-029, 2022. a
Davison, I. and Underhill, J. R.: Tectonics and Sedimentation in Extensional Rifts: Implications for Petroleum Systems, in: Tectonics and Sedimentation: Implications for Petroleum Systems, vol. 100, edited by: Gao, D., American Association of Petroleum Geologists, https://doi.org/10.1306/13351547M1001556, 2013. a
Debure, M., Lassin, A., Marty, N. C., Claret, F., Virgone, A., Calassou, S., and Gaucher, E. C.: Thermodynamic evidence of giant salt deposit formation by serpentinization: an alternative mechanism to solar evaporation, Sci. Rep.-UK, 9, 11720, https://doi.org/10.1038/s41598-019-48138-9, 2019. a
de Jager, J. and Geluk, M. C.: Petroleum geology, in: Geology of the Netherlands, edited by: Wong, T. E., Batjes, D. A. J., and de Jager, J., Royal Netherlands Academy of Arts and Sciences, 241–264, 2007. a
DeMets, C., Gordon, R. G., and Argus, D. F.: Geologically current plate motions, Geophys. J. Int., 181, 1–80, https://doi.org/10.1111/j.1365-246X.2009.04491.x, 2010. a
Depicker, A., Jacobs, L., Mboga, N., Smets, B., Van Rompaey, A., Lennert, M., Wolff, E., Kervyn, F., Michellier, C., Dewitte, O., and Govers, G.: Historical dynamics of landslide risk from population and forest-cover changes in the Kivu Rift, Nature Sustainability, 4, 965–974, https://doi.org/10.1038/s41893-021-00757-9, 2021. a, b, c
Dèzes, P., Schmid, S. M., and Ziegler, P. A.: Evolution of the European Cenozoic Rift System: interaction of the Alpine and Pyrenean orogens with their foreland lithosphere, Tectonophysics, 389, 1–33, https://doi.org/10.1016/j.tecto.2004.06.011, 2004. a
Dick, H. J. B., Lin, J., and Schouten, H.: An ultraslow-spreading class of ocean ridge, Nature, 426, 405–412, https://doi.org/10.1038/nature02128, 2003. a, b
Diessel, C. F. K.: Coal-Producing Tectonic Environments, in: Coal-Bearing Depositional Systems, edited by: Diessel, C. F. K., Springer Berlin Heidelberg, Berlin, Heidelberg, 515–595, https://doi.org/10.1007/978-3-642-75668-9_9, 1992. a, b
Dill, H. G.: A review of mineral resources in Malawi: With special reference to aluminium variation in mineral deposits, J. Afr. Earth Sci., 47, 153–173, https://doi.org/10.1016/j.jafrearsci.2006.12.006, 2007. a
Dill, H. G. and Ludwig, R.-R.: Geomorphological-sedimentological studies of landform types and modern placer deposits in the savanna (Southern Malawi), Ore Geol. Rev., 33, 411–434, https://doi.org/10.1016/j.oregeorev.2007.02.002, 2008. a
Dompnier, B., Luneau, J.-F., Phalip, B., and Flauraud, V.: Clermont. La cathédrale de pierre noire, haut-lieu symbolique de l'Auvergne à l'histoire prestigieuse, La Nuée bleue, https://uca.hal.science/hal-01651871 (last access: 11 July 2024), 2014. a
Doré, A. G., Lundin, E. R., Jensen, L. N., Birkeland, Ø., Eliassen, P. E., and Fichler, C.: Principal tectonic events in the evolution of the northwest European Atlantic margin, Geological Society, London, Petroleum Geology Conference Series, 5, 41–61, https://doi.org/10.1144/0050041, 1999. a, b
Doser, D. I.: Faulting within the eastern Baikal rift as characterized by earthquake studies, Tectonophysics, 196, 109–139, https://doi.org/10.1016/0040-1951(91)90292-Z, 1991. a
Duffy, O., Hudec, M., Peel, F., Apps, G., Bump, A., Moscardelli, L., Dooley, T., Fernandez, N., Bhattacharya, S., Wisian, K., and Shuster, M.: The Role of Salt Tectonics in the Energy Transition: An Overview and Future Challenges, τeκτoniκa, 1, 18–48, https://doi.org/10.55575/tektonika2023.1.1.11, 2023. a, b, c, d, e, f
Dumais, M.-A., Gernigon, L., Olesen, O., Johansen, S. E., and Brönner, M.: New interpretation of the spreading evolution of the Knipovich Ridge derived from aeromagnetic data, Geophys. J. Int., 224, 1422–1428, https://doi.org/10.1093/gji/ggaa527, 2020. a
Ebinger, C. J.: Tectonic development of the western branch of the East African rift system, GSA Bulletin, 101, 885–903, https://doi.org/10.1130/0016-7606(1989)101<0885:TDOTWB>2.3.CO;2, 1989. a
Einarsson, P., Hjartardóttir, Á. R., Hreinsdóttir, S., and Imsland, P.: The structure of seismogenic strike-slip faults in the eastern part of the Reykjanes Peninsula Oblique Rift, SW Iceland, J. Volcanol. Geoth. Res., 391, 106372, https://doi.org/10.1016/j.jvolgeores.2018.04.029, 2020. a
Elbarbary, S., Abdel Zaher, M., Saibi, H., Fowler, A.-R., and Saibi, K.: Geothermal renewable energy prospects of the African continent using GIS, Geothermal Energy, 10, 1–19, https://doi.org/10.1186/s40517-022-00219-1, 2022. a
Erratt, D., Thomas, G. M., and Wall, G. R. T.: The evolution of the Central North Sea Rift, Geological Society, London, Petroleum Geology Conference Series, 5, 63–82, https://doi.org/10.1144/0050063, 1999. a
EU: Critical Raw Materials: ensuring secure and sustainable supply chains for EU's green and digital future, European Commission, Press Corner, https://ec.europa.eu/commission/presscorner/detail/en/ip_23_1661 (last access: 11 July 2024), 2023. a
Fedorik, J., Delaunay, A., Losi, G., Panara, Y., Menegoni, N., Afifi, A. M., Arkadakskiy, S., Al Malallah, M., Oelkers, E., Gislason, S. R., Ahmed, Z., and Kunnummal, N.: Structure and fracture characterization of the Jizan group: Implications for subsurface CO2 basalt mineralization, Front. Earth Sci., 10, 946532, https://doi.org/10.3389/feart.2022.946532, 2023. a, b
Fielding, L., Najman, Y., Millar, I., Butterworth, P., Garzanti, E., Vezzoli, G., Barfod, D., and Kneller, B.: The initiation and evolution of the River Nile, Earth Planet. Sc. Lett., 489, 166–178, https://doi.org/10.1016/j.epsl.2018.02.031, 2018. a, b
Fine, I. V., Rabinovich, A. B., Bornhold, B. D., Thomson, R. E., and Kulikov, E. A.: The Grand Banks landslide-generated tsunami of November 18, 1929: preliminary analysis and numerical modeling, Mar. Geol., 215, 45–57, https://doi.org/10.1016/j.margeo.2004.11.007, 2005. a, b
Fleuchaus, P., Godschalk, B., Stober, I., and Blum, P.: Worldwide application of aquifer thermal energy storage – A review, Renew. Sust. Energ. Rev., 94, 861–876, https://doi.org/10.1016/j.rser.2018.06.057, 2018. a
Forel, B., Monna, F., Petit, C., Bruguier, O., Losno, R., Fluck, P., Begeot, C., Richard, H., Bichet, V., and Chateau, C.: Historical mining and smelting in the Vosges Mountains (France) recorded in two ombrotrophic peat bogs, J. Geochem. Explor., 107, 9–20, https://doi.org/10.1016/j.gexplo.2010.05.004, 2010. a
Fossen, H., Schultz, R. A., Rundhovde, E., Rotevatn, A., and Buckley, S. J.: Fault linkage and graben stepovers in the Canyonlands (Utah) and the North Sea Viking Graben, with implications for hydrocarbon migration and accumulation, AAPG Bull., 94, 597–613, https://doi.org/10.1306/10130909088, 2010. a
Franke, D.: Rifting, lithosphere breakup and volcanism: Comparison of magma-poor and volcanic rifted margins, Mar. Petrol. Geol., 43, 63–87, https://doi.org/10.1016/j.marpetgeo.2012.11.003, 2013. a, b, c, d, e
Franke, D., Hinz, K., and Oncken, O.: The Laptev Sea Rift, Mar. Petrol. Geol., 18, 1083–1127, https://doi.org/10.1016/S0264-8172(01)00041-1, 2001. a
Freymark, J., Sippel, J., Scheck-Wenderoth, M., Bär, K., Stiller, M., Fritsche, J.-G., and Kracht, M.: The deep thermal field of the Upper Rhine Graben, Tectonophysics, 694, 114–129, https://doi.org/10.1016/j.tecto.2016.11.013, 2017. a
Friedmann, S. J. and Burbank, D. W.: Rift basins and supradetachment basins: intracontinental extensional end-members, Basin Res., 7, 109–127, https://doi.org/10.1111/j.1365-2117.1995.tb00099.x, 1995. a
Frímann, J.: A short history of earthquake activity on South Icelandic Seismic Zone (SISZ), https://icelandgeology.net/?p=745 (last access: 23 October 2023), 2011. a
Fubelli, G. and Dramis, F.: Geo-hazard in Ethiopia, in: Landscapes and Landforms of Ethiopia, edited by: Billi, P., Springer Netherlands, Dordrecht, 351–367, https://doi.org/10.1007/978-94-017-8026-1_20, 2015. a, b
Fuchs, S., Hannington, M. D., and Petersen, S.: Divining gold in seafloor polymetallic massive sulfide systems, Miner. Deposita, 54, 789–820, https://doi.org/10.1007/s00126-019-00895-3, 2019. a
Gallagher, K., Brown, R., Osmaston, M., Ebinger, C., and Bishop, P.: Denudation and Uplift at Passive Margins: The Record on the Atlantic Margin of Southern Africa [and Discussion], Philos. T. Roy. Soc. A, 357, 835–859, 1999. a
Gasanzade, F., Witte, F., Tuschy, I., and Bauer, S.: Integration of geological compressed air energy storage into future energy supply systems dominated by renewable power sources, Energ. Convers. Manage., 277, 116643, https://doi.org/10.1016/j.enconman.2022.116643, 2023. a
Gaucher, E. C.: New Perspectives in the Industrial Exploration for Native Hydrogen, Elements, 16, 8–9, https://doi.org/10.2138/gselements.16.1.8, 2020. a, b
Gaucher, E. C., Moretti, I., Pélissier, N., Burridge, G., and Gonthier, N.: The place of natural hydrogen in the energy transition: A position paper, European Geologist, 55, 5–9, https://doi.org/10.5281/zenodo.8108239, 2023. a, b
Gawthorpe, R. L. and Leeder, M. R.: Tectono-sedimentary evolution of active extensional basins, Basin Res., 12, 195–218, https://doi.org/10.1111/j.1365-2117.2000.00121.x, 2000. a, b
Gayrin, P., Wrona, T., and Brune, S.: Semi-automated fault extraction and quantitative structural analysis from DEM data, a comprehensive tool for fault network analysis, EGU General Assembly 2023, Vienna, Austria, 24–28 Apr 2023, EGU23-12595, https://doi.org/10.5194/egusphere-egu23-12595, 2023. a
Geoscience Australia: Geoscience Australia, https://www.ga.gov.au/nopims, last access: 15 March 2024. a
Gernon, T. M., Jones, S. M., Brune, S., Hincks, T. K., Palmer, M. R., Schumacher, J. C., Primiceri, R. M., Field, M., Griffin, W. L., O'Reilly, S. Y., Keir, D., Spencer, C. J., Merdith, A. S., and Glerum, A.: Rift-induced disruption of cratonic keels drives kimberlite volcanism, Nature, 620, 344–350, https://doi.org/10.1038/s41586-023-06193-3, 2023. a
Glerum, A., Brune, S., Stamps, D. S., and Strecker, M. R.: Victoria continental microplate dynamics controlled by the lithospheric strength distribution of the East African Rift, Nat. Commun., 11, 2881, https://doi.org/10.1038/s41467-020-16176-x, 2020. a
Goitom, B., Oppenheimer, C., Hammond, J. O. S., Grandin, R., Barnie, T., Donovan, A., Ogubazghi, G., Yohannes, E., Kibrom, G., Kendall, J.-M., Carn, S. A., Fee, D., Sealing, C., Keir, D., Ayele, A., Blundy, J., Hamlyn, J., Wright, T., and Berhe, S.: First recorded eruption of Nabro volcano, Eritrea, 2011, B. Volcanol., 77, 85, https://doi.org/10.1007/s00445-015-0966-3, 2015. a
Goitom, B., Werner, M. J., Goda, K., Kendall, J.-M., Hammond, J. O. S., Ogubazghi, G., Oppenheimer, C., Helmstetter, A., Keir, D., and Illsley-Kemp, F.: Probabilistic Seismic-Hazard Assessment for Eritrea, B. Seismol. Soc. Am., 107, 1478–1494, https://doi.org/10.1785/0120160210, 2017. a
Gong, Y., Pease, V., Wang, H., Gan, H., Liu, E., Ma, Q., Zhao, S., and He, J.: Insights into evolution of a rift basin: Provenance of the middle Eocene-lower Oligocene strata of the Beibuwan Basin, South China Sea from detrital zircon, Sediment. Geol., 419, 105908, https://doi.org/10.1016/j.sedgeo.2021.105908, 2021. a
Goodfellow, W. D., Lydon, J. W., and Turner, R. J. W.: Geology and genesis of stratiform sediment-hosted (SEDEX) zinc-lead-silver sulphide deposits, Geol. Assoc. Can. Spec. Pap., 40, 201–252, 1993. a, b
Gouin, P.: Earthquake History of Ethiopia and the Horn of Africa, International Development Research Centre, Oiawa, Ontario, 258 pp., ISBN 0889361940, 1979. a, b, c
Gouiza, M. and Naliboff, J.: Rheological inheritance controls the formation of segmented rifted margins in cratonic lithosphere, Nat. Commun., 12, 4653, https://doi.org/10.1038/s41467-021-24945-5, 2021. a
Groves, D. I. and Bierlein, F. P.: Geodynamic settings of mineral deposit systems, J. Geol. Soc. London, 164, 19–30, https://doi.org/10.1144/0016-76492006-065, 2007. a, b
Gudmundsson, A.: Infrastructure and evolution of ocean-ridge discontinuities in Iceland, J. Geodyn., 43, 6–29, https://doi.org/10.1016/j.jog.2006.09.002, 2007. a
Gudmundsson, M. T., Thordarson, T., Höskuldsson, A., Larsen, G., Björnsson, H., Prata, F. J., Oddsson, B., Magnússon, E., Högnadóttir, T., Petersen, G. N., Hayward, C. L., Stevenson, J. A., and Jónsdóttir, I.: Ash generation and distribution from the April–May 2010 eruption of Eyjafjallajökull, Iceland, Sci. Rep.-UK, 2, 572, https://doi.org/10.1038/srep00572, 2012. a
Gunnell, Y. and Fleitout, L.: Shoulder uplift of the Western Ghats passive margin, India: a denudational model, Earth Surf. Proc. Land., 23, 391–404, https://doi.org/10.1002/(SICI)1096-9837(199805)23:5<391::AID-ESP853>3.0.CO;2-5, 1998. a
Gusiakov, V. K.: Tsunami impact on the African continent: historical cases and hazard evaluation, in: Extreme Natural Hazards, Disaster Risks and Societal Implications, Cambridge University Press, 225–233, https://doi.org/10.1017/CBO9781139523905.021, 2014. a, b
Hagemann, S. G., Lisitsin, V. A., and Huston, D. L.: Mineral system analysis: Quo vadis, Ore Geol. Rev., 76, 504–522, https://doi.org/10.1016/j.oregeorev.2015.12.012, 2016. a
Hand, E.: ECONOMIC GEOLOGY. Massive helium fields found in rift zone of Tanzania, Science, 353, 109–110, https://doi.org/10.1126/science.353.6295.109, 2016. a, b
Hannington, M. D., De Ronde, C. E. J., and Petersen, S.: Sea-Floor Tectonics and Submarine Hydrothermal Systems, in: One Hundredth Anniversary Volume, edited by: Hedenquist, J. W., Thompson, J. F. H., Goldfarb, R. J., and Richards, J. P., Society of Economic Geologists, https://doi.org/10.5382/AV100.06, 2005. a
Hansen, S. E., Nyblade, A. A., and Benoit, M. H.: Mantle structure beneath Africa and Arabia from adaptively parameterized P-wave tomography: Implications for the origin of Cenozoic Afro-Arabian tectonism, Earth Planet. Sc. Lett., 319-320, 23–34, https://doi.org/10.1016/j.epsl.2011.12.023, 2012. a
Hassan, R., Williams, S. E., Gurnis, M., and Müller, D.: East African topography and volcanism explained by a single, migrating plume, Geosci. Front., 11, 1669–1680, https://doi.org/10.1016/j.gsf.2020.01.003, 2020. a, b
Hauber, E., Grott, M., and Kronberg, P.: Martian rifts: Structural geology and geophysics, Earth Planet. Sc. Lett., 294, 393–410, https://doi.org/10.1016/j.epsl.2009.11.005, 2010. a
Hayward, N., Magnall, J. M., Taylor, M., King, R., McMillan, N., and Gleeson, S. A.: The Teena Zn-Pb Deposit (McArthur Basin, Australia). Part I: Syndiagenetic Base Metal Sulfide Mineralization Related to Dynamic Subbasin Evolution, Econ. Geol. Bull. Soc., 116, 1743–1768, https://doi.org/10.5382/econgeo.4846, 2021. a
Heap, M. J., Kushnir, A. R. L., Gilg, H. A., Wadsworth, F. B., Reuschlé, T., and Baud, P.: Microstructural and petrophysical properties of the Permo-Triassic sandstones (Buntsandstein) from the Soultz-sous-Forêts geothermal site (France), Geothermal Energy, 5, 1–37, https://doi.org/10.1186/s40517-017-0085-9, 2017. a
Hearn, G. J.: Some of the geological challenges and opportunities associated with the dynamics of the Cenozoic East African Rift System, Q. J. Eng. Geol. Hydroge., 55, qjegh2021-060, https://doi.org/10.1144/qjegh2021-060, 2022. a, b, c, d, e
Heidbach, O., Rajabi, M., Cui, X., Fuchs, K., Müller, B., Reinecker, J., Reiter, K., Tingay, M., Wenzel, F., Xie, F., Ziegler, M. O., Zoback, M.-L., and Zoback, M.: The World Stress Map database release 2016: Crustal stress pattern across scales, Tectonophysics, 744, 484–498, https://doi.org/10.1016/j.tecto.2018.07.007, 2018. a, b
Heinrich, C. A. and Candela, P. A.: 13.1 – Fluids and Ore Formation in the Earth's Crust, in: Treatise on Geochemistry, 2nd edn., edited by: Holland, H. D. and Turekian, K. K., Elsevier, Oxford, 1–28, https://doi.org/10.1016/B978-0-08-095975-7.01101-3, 2014. a, b, c
Hitzman, M. W., Selley, D., and Bull, S.: Formation of Sedimentary Rock-Hosted Stratiform Copper Deposits through Earth History, Econ. Geol. Bull. Soc., 105, 627–639, https://doi.org/10.2113/gsecongeo.105.3.627, 2010. a, b
Hoggard, M. J., Czarnota, K., Richards, F. D., Huston, D. L., Jaques, A. L., and Ghelichkhan, S.: Global distribution of sediment-hosted metals controlled by craton edge stability, Nat. Geosci., 13, 504–510, https://doi.org/10.1038/s41561-020-0593-2, 2020. a, b, c
Hosny, A., Omar, K., and Ali, S. M.: The Gulf of Suez earthquake, 30 January 2012, northeast of Egypt, Rend. Lincei-Sci. Fis., 24, 377–386, https://doi.org/10.1007/s12210-013-0244-2, 2013. a
Hosseinpour, M., Müller, R. D., Williams, S. E., and Whittaker, J. M.: Full-fit reconstruction of the Labrador Sea and Baffin Bay, Solid Earth, 4, 461–479, https://doi.org/10.5194/se-4-461-2013, 2013. a
Howell, J. A., Schwarz, E., Spalletti, L. A., and Veiga, G. D.: The Neuquén Basin: an overview, Geological Society, London, Special Publications, 252, 1–14, https://doi.org/10.1144/GSL.SP.2005.252.01.01, 2005. a
Hrubcová, P., Geissler, W. H., Bräuer, K., Vavryčuk, V., Tomek, Č., and Kämpf, H.: Active magmatic underplating in western Eger rift, central Europe, Tectonics, 36, 2846–2862, https://doi.org/10.1002/2017tc004710, 2017. a
Hurni, H., Abate, S., Bantider, A., Debele, B., Ludi, E., Portner, B., Yitaferu, B., and Zeleke, G.: Land degradation and sustainable land management in the Highlands of Ethiopia, in: Global Change and Sustainable Development: A Synthesis of Regional Experiences from Research Partnerships, vol. 5 of Perspectives/NCCR North-South, edited by: Hurni, H. and Wiesmann, U., Geographica Bernensia, Bern, 15, https://boris.unibe.ch/5959/ (last access: 11 July 2024), 2010. a
Husmo, T., Hamar, G. P., Høiland, O., Johannessen, E. P., Rømuld, A., Spencer, A. M., and Tiverton, R.: Lower and middle Jurassic, in: The Millennium Atlas: Petroleum Geology of the Central and Northern North Sea, edited by: Evans, D., Graham, C., Armour, A., and Bathurst, P., Geological Society, London, 191–211, 2003. a
IEA: The Role of Critical Minerals in Clean Energy Transitions, Tech. rep., IEA, https://www.iea.org/reports/the-role-of-critical-minerals-in-clean-energy-transitions (last access: 11 July 2024), 2021. a, b
IEA: Emissions from Oil and Gas Operations in Net Zero Transitions, Tech. rep., IEA, https://www.iea.org/reports/emissions-from-oil-and-gas-operations-in-net-zero-transitions (last access: 11 July 2024), 2023a. a
IEA: International Energy Outlook 2023, Tech. rep., IEA, https://www.eia.gov/outlooks/ieo/pdf/IEO2023_Release_Presentation.pdf (last access: 11 July 2024), 2023b. a
IHFC: International Heat Flow Commission (IHFC), https://ihfc-iugg.org/ (last access: 23 October 2023), 2023. a
Illsley-Kemp, F., Keir, D., Bull, J. M., Gernon, T. M., Ebinger, C., Ayele, A., Hammond, J. O. S., Kendall, J.-M., Goitom, B., and Belachew, M.: Seismicity During Continental Breakup in the Red Sea Rift of Northern Afar, J. Geophys. Res.-Sol. Ea., 123, 2345–2362, https://doi.org/10.1002/2017JB014902, 2018. a
IPCC: AR6 Synthesis Report: Climate Change 2023, Tech. rep., IPCC, https://www.ipcc.ch/report/sixth-assessment-report-cycle/ (last access: 11 July 2024), 2023. a
IRENA: Geothermal development in Eastern Africa, Tech. rep., IRENA, https://www.irena.org/publications/2020/Nov/Geothermal-development-in-Eastern-Africa (last access: 11 July 2024), 2020. a, b
Ismail, E. H. and Abdelsalam, M. G.: Morpho-tectonic analysis of the Tekeze River and the Blue Nile drainage systems on the Northwestern Plateau, Ethiopia, J. Afr. Earth Sci., 69, 34–47, https://doi.org/10.1016/j.jafrearsci.2012.04.005, 2012. a
Jacobs, L., Dewitte, O., Poesen, J., Maes, J., Mertens, K., Sekajugo, J., and Kervyn, M.: Landslide characteristics and spatial distribution in the Rwenzori Mountains, Uganda, J. Afr. Earth Sci., 134, 917–930, https://doi.org/10.1016/j.jafrearsci.2016.05.013, 2017. a
Jacobs, L., Dewitte, O., Poesen, J., Sekajugo, J., Nobile, A., Rossi, M., Thiery, W., and Kervyn, M.: Field-based landslide susceptibility assessment in a data-scarce environment: the populated areas of the Rwenzori Mountains, Nat. Hazards Earth Syst. Sci., 18, 105–124, https://doi.org/10.5194/nhess-18-105-2018, 2018. a
Jefferson, C. W. and Delaney, G.: EXTECH IV: Geology and Uranium EXploration TECHnology of the Proterozoic Athabasca Basin, Saskatchewan and Alberta, Tech. rep., Geological Survey of Canada, Bulletin, https://publications.gc.ca/site/eng/9.617310/publication.html (last access: 11 July 2024), 2007. a
Jelsma, H., Barnett, W., Richards, S., and Lister, G.: Tectonic setting of kimberlites, Lithos, 112, 155–165, https://doi.org/10.1016/j.lithos.2009.06.030, 2009. a
Johansson, L., Zahirovic, S., and Müller, R. D.: The interplay between the eruption and weathering of large igneous provinces and the deep-time carbon cycle, Geophys. Res. Lett., 45, 5380–5389, https://doi.org/10.1029/2017gl076691, 2018. a
Jolie, E., Scott, S., Faulds, J., Chambefort, I., Axelsson, G., Gutiérrez-Negrín, L. C., Regenspurg, S., Ziegler, M., Ayling, B., Richter, A., and Zemedkun, M. T.: Geological controls on geothermal resources for power generation, Nature Reviews Earth & Environment, 2, 324–339, https://doi.org/10.1038/s43017-021-00154-y, 2021. a
Jones, N.: How dangerous is Africa's explosive Lake Kivu?, https://www.nature.com/immersive/d41586-021-02523-5/index.html (last access: 23 October 2023), 2021. a, b
Jun, Y.-S., Zhang, L., Min, Y., and Li, Q.: Nanoscale Chemical Processes Affecting Storage Capacities and Seals during Geologic CO2 Sequestration, Accounts Chem. Res., 50, 1521–1529, https://doi.org/10.1021/acs.accounts.6b00654, 2017. a
Kamenetsky, V. S., Doroshkevich, A. G., Elliott, H. A. L., and Zaitsev, A. N.: Carbonatites: Contrasting, Complex, and Controversial, Elements, 17, 307–314, https://doi.org/10.2138/gselements.17.5.307, 2021. a
Kapetanidis, V., Karakonstantis, A., Papadimitriou, P., Pavlou, K., Spingos, I., Kaviris, G., and Voulgaris, N.: The 19 July 2019 earthquake in Athens, Greece: A delayed major aftershock of the 1999 Mw=6.0 event, or the activation of a different structure?, J. Geodyn., 139, 101766, https://doi.org/10.1016/j.jog.2020.101766, 2020. a
Karstens, J., Preine, J., Crutchley, G. J., Kutterolf, S., van der Bilt, W. G. M., Hooft, E. E. E., Druitt, T. H., Schmid, F., Cederstrøm, J. M., Hübscher, C., Nomikou, P., Carey, S., Kühn, M., Elger, J., and Berndt, C.: Revised Minoan eruption volume as benchmark for large volcanic eruptions, Nat. Commun., 14, 2497, https://doi.org/10.1038/s41467-023-38176-3, 2023. a
Katz, B. J.: A survey of rift basin source rocks, Geological Society, London, Special Publications, 80, 213–240, https://doi.org/10.1144/GSL.SP.1995.080.01.11, 1995. a
Keller, G. R. and Stephenson, R. A.: The Southern Oklahoma and Dniepr-Donets aulacogens: A comparative analysis, in: 4-D Framework of Continental Crust, vol. 200, edited by: Hatcher Jr., R. D., Carlson, M. P., McBride, J. H., and Catalán, J. R. M., Geological Society of America, https://doi.org/10.1130/2007.1200(08), 2007. a
Kellogg, L. H., Lokavarapu, H., and Turcotte, D. L.: Carbonation and the Urey reaction, Am. Mineral., 104, 1365–1368, https://doi.org/10.2138/am-2019-6880, 2019. a, b
Kelman, I., Alexander, D., Fearnley, C., Jenkins, S., and Sammonds, P.: Systemic risks perspectives of Eyjafjallajökull volcano's 2010 eruption, Progress in Disaster Science, 18, 100282, https://doi.org/10.1016/j.pdisas.2023.100282, 2023. a
Khlystov, O. M., Poort, J., Mazzini, A., Akhmanov, G. G., Minami, H., Hachikubo, A., Khabuev, A. V., Kazakov, A. V., De Batist, M., Naudts, L., Chensky, A. G., and Vorobeva, S. S.: Shallow-rooted mud volcanism in Lake Baikal, Mar. Petrol. Geol., 102, 580–589, https://doi.org/10.1016/j.marpetgeo.2019.01.005, 2019. a
Kim, S., Dusseault, M., Babarinde, O., and Wickens, J.: Compressed air energy storage (CAES): current status, geomechanical aspects and future opportunities, Geological Society, London, Special Publications, 528, 87–100, https://doi.org/10.1144/SP528-2022-54, 2023. a, b
Kober, T., Schiffer, H.-W., Densing, M., and Panos, E.: Global energy perspectives to 2060 – WEC's World Energy Scenarios 2019, Energy Strateg. Rev., 31, 100523, https://doi.org/10.1016/j.esr.2020.100523, 2020. a
Kodikara, G. R. L., Woldai, T., van Ruitenbeek, F. J. A., Kuria, Z., van der Meer, F., Shepherd, K. D., and van Hummel, G. J.: Hyperspectral remote sensing of evaporate minerals and associated sediments in Lake Magadi area, Kenya, Int. J. Appl. Earth Obs., 14, 22–32, https://doi.org/10.1016/j.jag.2011.08.009, 2012. a, b, c
Kölbel, L., Kölbel, T., Herrmann, L., Kaymakci, E., Ghergut, I., Poirel, A., and Schneider, J.: Lithium extraction from geothermal brines in the Upper Rhine Graben: A case study of potential and current state of the art, Hydrometallurgy, 221, 106131, https://doi.org/10.1016/j.hydromet.2023.106131, 2023. a, b
Koppers, A. A. P. and Coggon, R.: Digital collections: Exploring Earth by Scientific Ocean Drilling, UC San Diego Library Digital Collections, https://doi.org/10.6075/J0W66J9H, 2020. a
Korup, O.: Earth's portfolio of extreme sediment transport events, Earth-Sci. Rev., 112, 115–125, https://doi.org/10.1016/j.earscirev.2012.02.006, 2012. a, b
Kropáček, J., Vařilová, Z., Baroň, I., Bhattacharya, A., Eberle, J., and Hochschild, V.: Remote Sensing for Characterisation and Kinematic Analysis of Large Slope Failures: Debre Sina Landslide, Main Ethiopian Rift Escarpment, Remote Sens.-Basel, 7, 16183–16203, https://doi.org/10.3390/rs71215821, 2015. a
Kudrass, H., Wood, R., and Falconer, R.: Submarine Phosphorites: The Deposits of the Chatham Rise, New Zealand, off Namibia and Baja California, Mexico – Origin, Exploration, Mining, and Environmental Issues, in: Deep-Sea Mining: Resource Potential, Technical and Environmental Considerations, edited by: Sharma, R., Springer International Publishing, Cham, 165–187, https://doi.org/10.1007/978-3-319-52557-0_5, 2017. a
Kung, A., Svobodova, K., Lèbre, E., Valenta, R., Kemp, D., and Owen, J. R.: Governing deep sea mining in the face of uncertainty, J. Environ. Manage., 279, 111593, https://doi.org/10.1016/j.jenvman.2020.111593, 2021. a
Kydonakis, K., Brun, J.-P., and Sokoutis, D.: North Aegean core complexes, the gravity spreading of a thrust wedge, J. Geophys. Res.-Sol. Ea., 120, 595–616, https://doi.org/10.1002/2014JB011601, 2015. a
La Rosa, A., Pagli, C., Keir, D., Sani, F., Corti, G., Wang, H., and Possee, D.: Observing oblique slip during rift linkage in northern afar, Geophys. Res. Lett., 46, 10782–10790, https://doi.org/10.1029/2019gl084801, 2019. a
Lavier, L. L. and Manatschal, G.: A mechanism to thin the continental lithosphere at magma-poor margins, Nature, 440, 324–328, https://doi.org/10.1038/nature04608, 2006. a
Lawley, C. J. M., McCafferty, A. E., Graham, G. E., Huston, D. L., Kelley, K. D., Czarnota, K., Paradis, S., Peter, J. M., Hayward, N., Barlow, M., Emsbo, P., Coyan, J., San Juan, C. A., and Gadd, M. G.: Data–driven prospectivity modelling of sediment–hosted Zn–Pb mineral systems and their critical raw materials, Ore Geol. Rev., 141, 104635, https://doi.org/10.1016/j.oregeorev.2021.104635, 2022. a, b
Leach, D. L., Bradley, D. C., Huston, D., Pisarevsky, S. A., Taylor, R. D., and Gardoll, S. J.: Sediment-Hosted Lead-Zinc Deposits in Earth History, Econ. Geol. Bull. Soc., 105, 593–625, https://doi.org/10.2113/gsecongeo.105.3.593, 2010. a, b, c
Ledésert, B. A., Hébert, R. L., Mouchot, J., Bosia, C., Ravier, G., Seibel, O., Dalmais, É., Ledésert, M., Trullenque, G., Sengelen, X., and Genter, A.: Scaling in a Geothermal Heat Exchanger at Soultz-Sous-Forêts (Upper Rhine Graben, France): A XRD and SEM-EDS Characterization of Sulfide Precipitates, Geosci. J., 11, 271, https://doi.org/10.3390/geosciences11070271, 2021. a
Lee, K. C.: Classification of geothermal resources by exergy, Geothermics, 30, 431–442, https://doi.org/10.1016/S0375-6505(00)00056-0, 2001. a
Leeder, M. R., Harris, T., and Kirkby, M. J.: Sediment supply and climate change: implications for basin stratigraphy, Basin Res., 10, 7–18, https://doi.org/10.1046/j.1365-2117.1998.00054.x, 1998. a
Lefeuvre, N., Truche, L., Donzé, F.-V., Gal, F., Tremosa, J., Fakoury, R.-A., Calassou, S., and Gaucher, E. C.: Natural hydrogen migration along thrust faults in foothill basins: The North Pyrenean Frontal Thrust case study, Appl. Geochem., 145, 105396, https://doi.org/10.1016/j.apgeochem.2022.105396, 2022. a
Leifsson, Á.: Industrial use of the high-temperature geothermal field at Theistareykir, Iceland, Geothermics, 21, 631–640, https://doi.org/10.1016/0375-6505(92)90016-3, 1992. a
Levell, B. and Bowman, M.: The Petroleum Geology of NW Europe: 50 years of learning – a platform for present value and future success. An overview, Geological Society, London, Petroleum Geology Conference Series, 8, 1–8, https://doi.org/10.1144/PGC8.41, 2018. a
Leynaud, D., Mienert, J., and Vanneste, M.: Submarine mass movements on glaciated and non-glaciated European continental margins: A review of triggering mechanisms and preconditions to failure, Mar. Petrol. Geol., 26, 618–632, https://doi.org/10.1016/j.marpetgeo.2008.02.008, 2009. a, b
Li, Q., Xing, H., Liu, J., and Liu, X.: A review on hydraulic fracturing of unconventional reservoir, Petroleum, 1, 8–15, https://doi.org/10.1016/j.petlm.2015.03.008, 2015. a
Limberger, J., Boxem, T., Pluymaekers, M., Bruhn, D., Manzella, A., Calcagno, P., Beekman, F., Cloetingh, S., and van Wees, J.-D.: Geothermal energy in deep aquifers: A global assessment of the resource base for direct heat utilization, Renew. Sust. Energ. Rev., 82, 961–975, https://doi.org/10.1016/j.rser.2017.09.084, 2018. a
Lithgow-Bertelloni, C. and Silver, P. G.: Dynamic topography, plate driving forces and the African superswell, Nature, 395, 269–272, https://doi.org/10.1038/26212, 1998. a
Littke, R., Bayer, U., Dirk, G., and Nelskamp, S. (Eds.): Dynamics of Complex Intracontinental Basins, Springer Berlin, Heidelberg, https://doi.org/10.1007/978-3-540-85085-4, 2008. a
Liu, M. and Furlong, K. P.: Intrusion and underplating of mafic magmas: thermal-rheological effects and implications for Tertiary tectonomagmatism in the North American Cordillera, Tectonophysics, 237, 175–187, https://doi.org/10.1016/0040-1951(94)90253-4, 1994. a
Liu, M., Yang, Y., Shen, Z., Wang, S., Wang, M., and Wan, Y.: Active tectonics and intracontinental earthquakes in China: The kinematics and geodynamics, in: Continental Intraplate Earthquakes: Science, Hazard, and Policy Issues, vol. 425, edited by: Stein, S. and Mazzotti, S., Geological Society of America, https://doi.org/10.1130/2007.2425(19), 2007. a, b, c
Liu, Z., Perez-Gussinye, M., García-Pintado, J., Mezri, L., and Bach, W.: Mantle serpentinization and associated hydrogen flux at North Atlantic magma-poor rifted margins, Geology, 51, 284–289, https://doi.org/10.1130/G50722.1, 2023. a, b, c
Løvholt, F., Schulten, I., Mosher, D., Harbitz, C., and Krastel, S.: Modelling the 1929 Grand Banks slump and landslide tsunami, Geological Society, London, Special Publications, 477, 315–331, https://doi.org/10.1144/SP477.28, 2019. a
Ludden, J.: Where is geoscience going?, Geological Society, London, Special Publications, 499, 69–77, https://doi.org/10.1144/SP499-2019-212, 2020. a
Macdonald, K. C.: Mid-Ocean Ridge Tectonics, Volcanism, and Geomorphology, in: Encyclopedia of Ocean Sciences, 3rd edn., edited by: Cochran, J. K., Bokuniewicz, H. J., and Yager, P. L., Academic Press, Oxford, 405–419, https://doi.org/10.1016/B978-0-12-409548-9.11065-6, 2019. a, b, c, d
Mackay, A. W.: The paleoclimatology of Lake Baikal: A diatom synthesis and prospectus, Earth-Sci. Rev., 82, 181–215, https://doi.org/10.1016/j.earscirev.2007.03.002, 2007. a
Maestrelli, D., Montanari, D., Corti, G., Del Ventisette, C., Moratti, G., and Bonini, M.: Exploring the interactions between rift propagation and inherited crustal fabrics through experimental modeling, Tectonics, 39, e2020TC006211, https://doi.org/10.1029/2020tc006211, 2020. a, b, c, d
Maestrelli, D., Brune, S., Corti, G., Keir, D., Muluneh, A. A., and Sani, F.: Analog and numerical modeling of rift-rift-rift triple junctions, Tectonics, 41, e2022TC007491, https://doi.org/10.1029/2022tc007491, 2022. a
Magnall, J. M., Gleeson, S. A., and Paradis, S.: A new Subseafloor Replacement Model for the Macmillan Pass Clastic-Dominant Zn-Pb ± Ba Deposits (Yukon, Canada), Econ. Geol. Bull. Soc., 115, 953–959, https://doi.org/10.5382/econgeo.4719, 2020. a
Magoon, L. B. and Dow, W. G.: The Petroleum System – From Source to Trap, American Association of Petroleum Geologists, https://doi.org/10.1306/M60585, 1994. a
Manatschal, G., Chenin, P., Ulrich, M., Petri, B., Morin, M., and Ballay, M.: Tectono-magmatic evolution during the extensional phase of a Wilson Cycle: a review of the Alpine Tethys case and implications for Atlantic-type margins, Ital. J. Geosci., 142, 5–27, https://doi.org/10.3301/IJG.2023.01, 2023. a
Mancini, S., Segou, M., Werner, M. J., Parsons, T., Beroza, G., and Chiaraluce, L.: On the Use of High-Resolution and Deep-Learning Seismic Catalogs for Short-Term Earthquake Forecasts: Potential Benefits and Current Limitations, J. Geophys. Res.-Sol. Ea., 127, e2022JB025202, https://doi.org/10.1029/2022JB025202, 2022. a
Martin-Jones, C., Lane, C., Daele, M., Meeren, T. V. D., Wolff, C., Moorhouse, H., Tomlinson, E., and Verschuren, D.: History of scoria-cone eruptions on the eastern shoulder of the Kenya–Tanzania Rift revealed in the 250 ka sediment record of Lake Chala near Mount Kilimanjaro, J. Quaternary Sci., 35, 245–255, https://doi.org/10.1002/jqs.3140, 2020. a, b
Martínek, K., Verner, K., Hroch, T., Megerssa, L. A., Kopačková, V., Buriánek, D., Muluneh, A., Kalinová, R., Yakob, M., and Kassa, M.: Main Ethiopian Rift landslides formed in contrasting geological settings and climatic conditions, Nat. Hazards Earth Syst. Sci., 21, 3465–3487, https://doi.org/10.5194/nhess-21-3465-2021, 2021. a, b
Martins-Neto, M. A. and Catuneanu, O.: Rift sequence stratigraphy, Mar. Petrol. Geol., 27, 247–253, https://doi.org/10.1016/j.marpetgeo.2009.08.001, 2010. a
Masini, E., Manatschal, G., and Mohn, G.: The Alpine Tethys rifted margins: Reconciling old and new ideas to understand the stratigraphic architecture of magma-poor rifted margins, Sedimentology, 60, 174–196, https://doi.org/10.1111/sed.12017, 2013. a
Matter, J. M. and Kelemen, P. B.: Permanent storage of carbon dioxide in geological reservoirs by mineral carbonation, Nat. Geosci., 2, 837–841, https://doi.org/10.1038/ngeo683, 2009. a, b, c
Maystrenko, Y. P., Brönner, M., Olesen, O., Saloranta, T. M., and Slagstad, T.: Atmospheric precipitation and anomalous upper mantle in relation to intraplate seismicity in Norway, Tectonics, 39, e2020TC006070, https://doi.org/10.1029/2020tc006070, 2020. a
Mazzini, I., Cronin, T. M., Gawthorpe, R. L., Ll Collier, R. E., de Gelder, G., Golub, A. R., Toomey, M. R., Poirier, R. K., May Huang, H.-H., Phillips, M. P., McNeill, L. C., and Shillington, D. J.: A new deglacial climate and sea-level record from 20 to 8 ka from IODP381 site M0080, Alkyonides Gulf, eastern Mediterranean, Quaternary Sci. Rev., 313, 108192, https://doi.org/10.1016/j.quascirev.2023.108192, 2023. a
McFall, B. C. and Fritz, H. M.: Physical modelling of tsunamis generated by three-dimensional deformable granular landslides on planar and conical island slopes, P. Roy. Soc. A-Math. Phy., 472, 20160052, https://doi.org/10.1098/rspa.2016.0052, 2016. a
McKenzie, D.: Some remarks on the development of sedimentary basins, Earth Planet. Sc. Lett., 40, 25–32, https://doi.org/10.1016/0012-821X(78)90071-7, 1978. a
McNeill, L. C., Shillington, D. J., Carter, G. D. O., Everest, J. D., Gawthorpe, R. L., Miller, C., Phillips, M. P., Collier, R. E. L., Cvetkoska, A., De Gelder, G., Diz, P., Doan, M.-L., Ford, M., Geraga, M., Gillespie, J., Hemelsdaël, R., Herrero-Bervera, E., Ismaiel, M., Janikian, L., Kouli, K., Le Ber, E., Li, S., Maffione, M., Mahoney, C., Machlus, M. L., Michas, G., Nixon, C. W., Oflaz, S. A., Omale, A. P., Panagiotopoulos, K., Pechlivanidou, S., Sauer, S., Seguin, J., Sergiou, S., Zakharova, N. V., and Green, S.: High-resolution record reveals climate-driven environmental and sedimentary changes in an active rift, Sci. Rep.-UK, 9, 3116, https://doi.org/10.1038/s41598-019-40022-w, 2019. a
Meghraoui, M., Delouis, B., Ferry, M., Giardini, D., Huggenberger, P., Spottke, I., and Granet, M.: Active normal faulting in the upper Rhine graben and paleoseismic identification of the 1356 Basel earthquake, Science, 293, 2070–2073, https://doi.org/10.1126/science.1010618, 2001. a
Melcher, F., Grum, W., Simon, G., Thalhammer, T. V., and Stumpfl, E. F.: Petrogenesis of the Ophiolitic Giant Chromite Deposits of Kempirsai, Kazakhstan: a Study of Solid and Fluid Inclusions in Chromite, J. Petrol., 38, 1419–1458, https://doi.org/10.1093/petroj/38.10.1419, 1997. a
Merle, O.: A simple continental rift classification, Tectonophysics, 513, 88–95, https://doi.org/10.1016/j.tecto.2011.10.004, 2011. a
Merle, O., Boivin, P., Langlois, E., de Larouzière, F.-D., Michelin, Y., and Olive, C.: The UNESCO World Heritage Site of the Chaîne des Puys–Limagne Fault Tectonic Arena (Auvergne, France), Geosci. J., 13, 198, https://doi.org/10.3390/geosciences13070198, 2023. a, b
Micallef, A., Person, M., Berndt, C., Bertoni, C., Cohen, D., Dugan, B., Evans, R., Haroon, A., Hensen, C., Jegen, M., Key, K., Kooi, H., Liebetrau, V., Lofi, J., Mailloux, B. J., Martin-Nagle, R., Michael, H. A., Müller, T., Schmidt, M., Schwalenberg, K., Trembath-Reichert, E., Weymer, B., Zhang, Y., and Thomas, A. T.: Offshore Freshened Groundwater in Continental Margins, Rev. Geophys., 59, e2020RG000706, https://doi.org/10.1029/2020RG000706, 2021. a, b
Midzi, V., Hlatywayo, D. J., Chapola, L. S., Kebede, F., Atakan, K., Lombe, D. K., Turyomurugyendo, G., and Tugume, F. A.: Seismic hazard assessment in Eastern and Southern Africa, Ann. Geophys., 42, 1067–1083, https://doi.org/10.4401/ag-3770, 1999. a, b
Miller, A. R., Densmore, C. D., Degens, E. T., Hathaway, J. C., Manheim, F. T., McFarlin, P. F., Pocklington, R., and Jokela, A.: Hot brines and recent iron deposits in deeps of the Red Sea, Geochim. Cosmochim. Ac., 30, 341–359, https://doi.org/10.1016/0016-7037(66)90007-X, 1966. a
Mitali, J., Dhinakaran, S., and Mohamad, A. A.: Energy storage systems: a review, Energy Storage and Saving, 1, 166–216, https://doi.org/10.1016/j.enss.2022.07.002, 2022. a
Mohamed, G.-E. A. and Abd El-Aal, A. K.: Present Kinematic Regime and Recent Seismicity of Gulf Suez, Egypt, Geotectonics/Geotektonika, 52, 134–150, https://doi.org/10.1134/S0016852118010119, 2018. a
Mohr, P.: Ethiopian flood basalt province, Nature, 303, 577–584, https://doi.org/10.1038/303577a0, 1983. a
Mohriak, W., Nemčok, M., and Enciso, G.: South Atlantic divergent margin evolution: rift-border uplift and salt tectonics in the basins of SE Brazil, Geological Society, London, Special Publications, 294, 365–398, https://doi.org/10.1144/SP294.19, 2008. a
Mondal, S. K. and Mathez, E. A.: Origin of the UG2 chromitite layer, Bushveld Complex, J. Petrol., 48, 495–510, https://doi.org/10.1093/petrology/egl069, 2007. a
Moore, T. A.: Coalbed methane: A review, Int. J. Coal Geol., 101, 36–81, https://doi.org/10.1016/j.coal.2012.05.011, 2012. a
Morley, C. K.: Major unconformities/termination of extension events and associated surfaces in the South China Seas: Review and implications for tectonic development, J. Asian Earth Sci., 120, 62–86, https://doi.org/10.1016/j.jseaes.2016.01.013, 2016. a
Mudd, G. M., Jowitt, S. M., and Werner, T. T.: The world's lead-zinc mineral resources: Scarcity, data, issues and opportunities, Ore Geol. Rev., 80, 1160–1190, https://doi.org/10.1016/j.oregeorev.2016.08.010, 2017. a
Mukuhira, Y., Asanuma, H., Niitsuma, H., Schanz, U., and Häring, M.: Characterization of microseismic events with larger magnitude collected at Basel, Switzerland in 2006, Transactions – Geothermal Resources Council, 32, 74–80, 2008. a
Müller, R. D., Zahirovic, S., Williams, S. E., Cannon, J., Seton, M., Bower, D. J., Tetley, M. G., Heine, C., Le Breton, E., Liu, S., Russell, S. H. J., Yang, T., Leonard, J., and Gurnis, M.: A Global Plate Model Including Lithospheric Deformation Along Major Rifts and Orogens Since the Triassic, Tectonics, 38, 1884–1907, https://doi.org/10.1029/2018TC005462, 2019. a
Muther, T., Qureshi, H. A., Syed, F. I., Aziz, H., Siyal, A., Dahaghi, A. K., and Negahban, S.: Unconventional hydrocarbon resources: geological statistics, petrophysical characterization, and field development strategies, Journal of Petroleum Exploration and Production Technology, 12, 1463–1488, https://doi.org/10.1007/s13202-021-01404-x, 2022. a, b
Naliboff, J. and Buiter, S. J. H.: Rift reactivation and migration during multiphase extension, Earth Planet. Sc. Lett., 421, 58–67, https://doi.org/10.1016/j.epsl.2015.03.050, 2015. a
National Research Council: Selling the Nation's Helium Reserve, Tech. rep., National Research Council, Division on Engineering and Physical Sciences, National Materials Advisory Board, Board on Physics and Astronomy, Committee on Understanding the Impact of Selling the Helium Reserve, https://doi.org/10.17226/12844, 2010. a
Neely, J. S. and Stein, S.: Why do continental normal fault earthquakes have smaller maximum magnitudes?, Tectonophysics, 809, 228854, https://doi.org/10.1016/j.tecto.2021.228854, 2021. a, b
Nemčok, M.: Rifts and Passive Margins: Structural Architecture, Thermal Regimes, and Petroleum Systems, Cambridge University Press, https://doi.org/10.1017/CBO9781139198844, 2016. a, b, c, d
Neuharth, D., Brune, S., Wrona, T., Glerum, A., Braun, J., and Yuan, X.: Evolution of rift systems and their fault networks in response to surface processes, Tectonics, 41, e2021TC007166, https://doi.org/10.1029/2021tc007166, 2022. a, b
Novikova, T., Papadopoulos, G. A., and McCoy, F. W.: Modelling of tsunami generated by the giant Late Bronze Age eruption of Thera, South Aegean Sea, Greece, Geophys. J. Int., 186, 665–680, https://doi.org/10.1111/j.1365-246X.2011.05062.x, 2011. a
Nowell, D. A. G., Jones, M. C., and Pyle, D. M.: Episodic Quaternary volcanism in France and Germany, J. Quaternary Sci., 21, 645–675, https://doi.org/10.1002/jqs.1005, 2006. a, b
NPD: Faktasider – OD, https://factpages.npd.no/en (last access: 15 March 2024), 2024a. a
NPD: The Diskos National Data Repository (NDR), https://www.npd.no/en/diskos/ (last access: 15 March 2024), 2024b. a
Nyland, A. J., Walker, J., and Warren, G.: Evidence of the Storegga tsunami 8200 BP? An archaeological review of impact after a large-scale marine event in Mesolithic northern Europe, Front. Earth Sci., 9, 767460, https://doi.org/10.3389/feart.2021.767460, 2021. a
Okoko, G. O. and Olaka, L. A.: Can East African rift basalts sequester CO2? Case study of the Kenya rift, Scientific African, 13, e00924, https://doi.org/10.1016/j.sciaf.2021.e00924, 2021. a
Olajire, A. A.: A review of mineral carbonation technology in sequestration of CO2, J. Petrol. Sci. Eng., 109, 364–392, https://doi.org/10.1016/j.petrol.2013.03.013, 2013. a
Olierook, H. K. H., Fougerouse, D., Doucet, L. S., Liu, Y., Rayner, M. J., Danišík, M., Condon, D. J., McInnes, B. I. A., Jaques, A. L., Evans, N. J., McDonald, B. J., Li, Z.-X., Kirkland, C. L., Mayers, C., and Wingate, M. T. D.: Emplacement of the Argyle diamond deposit into an ancient rift zone triggered by supercontinent breakup, Nat. Commun., 14, 5274, https://doi.org/10.1038/s41467-023-40904-8, 2023. a
Olive, J.-A., Behn, M. D., and Malatesta, L. C.: Modes of extensional faulting controlled by surface processes, Geophys. Res. Lett., 41, 6725–6733, https://doi.org/10.1002/2014gl061507, 2014. a
Osman, A. I., Mehta, N., Elgarahy, A. M., Hefny, M., Al-Hinai, A., Al-Muhtaseb, A. H., and Rooney, D. W.: Hydrogen production, storage, utilisation and environmental impacts: a review, Environ. Chem. Lett., 20, 153–188, https://doi.org/10.1007/s10311-021-01322-8, 2022. a
Osselin, F., Soulaine, C., Fauguerolles, C., Gaucher, E. C., Scaillet, B., and Pichavant, M.: Orange hydrogen is the new green, Nat. Geosci., 15, 765–769, https://doi.org/10.1038/s41561-022-01043-9, 2022. a
Oth, A., Barrière, J., d'Oreye, N., Mavonga, G., Subira, J., Mashagiro, N., Kafundu, B., Fiama, S., Celli, G., de Dieu Birigande, J., Ntenge, A. J., and Kervyn, F.: Kivu Seismological Network (KivuSnet), https://doi.org/10.14470/XI058335, 2013. a
Pacini, N. and Harper, D. M.: Hydrological characteristics and water resources management in the Nile Basin, Ecohydrology & Hydrobiology, 16, 242–254, https://doi.org/10.1016/j.ecohyd.2016.09.001, 2016. a
Pagli, C., Wright, T. J., Ebinger, C. J., Yun, S.-H., Cann, J. R., Barnie, T., and Ayele, A.: Shallow axial magma chamber at the slow-spreading Erta Ale Ridge, Nat. Geosci., 5, 284–288, https://doi.org/10.1038/ngeo1414, 2012. a
Pagneux, E., Gudmundsson, M., Karlsdóttir, S., and Roberts, M.: Volcanogenic floods in Iceland: An assessment of hazards and risks at Öræfajökull and on the Markarfljót outwash plain, IMO, IES-UI, NCIP-DCPEM, ISBN 9789979997573, 2015. a
Papanastassiou, D., Gaki-Papanastassiou, K., and Maroukian, H.: Recognition of past earthquakes along the Sparta fault (Peloponnesus, southern Greece) during the Holocene, by combining results of different dating techniques, J. Geodyn., 40, 189–199, https://doi.org/10.1016/j.jog.2005.07.015, 2005. a
Pasquet, G., Houssein Hassan, R., Sissmann, O., Varet, J., and Moretti, I.: An Attempt to Study Natural H2 Resources across an Oceanic Ridge Penetrating a Continent: The Asal–Ghoubbet Rift (Republic of Djibouti), Geosci. J., 12, 16, https://doi.org/10.3390/geosciences12010016, 2021. a
Peace, A. L., Phethean, J. J. J., Franke, D., Foulger, G. R., Schiffer, C., Welford, J. K., McHone, G., Rocchi, S., Schnabel, M., and Doré, A. G.: A review of Pangaea dispersal and Large Igneous Provinces – In search of a causative mechanism, Earth-Sci. Rev., 206, 102902, https://doi.org/10.1016/j.earscirev.2019.102902, 2020. a, b
Peel, F. J.: How do salt withdrawal minibasins form? Insights from forward modelling, and implications for hydrocarbon migration, Tectonophysics, 630, 222–235, https://doi.org/10.1016/j.tecto.2014.05.027, 2014. a
Pérez-Gussinyé, M., Collier, J. S., Armitage, J. J., Hopper, J. R., Sun, Z., and Ranero, C. R.: Towards a process-based understanding of rifted continental margins, Nature Reviews Earth & Environment, 4, 166–184, https://doi.org/10.1038/s43017-022-00380-y, 2023. a
Peron-Pinvidic, G.: Rifting and Rifted Margins: Definitions, in: Continental Rifted Margins, edited by: Peron-Pinvidic, G., Wiley, 1–176, https://doi.org/10.1002/9781119986928.part1, 2022. a, b
Péron-Pinvidic, G. and Manatschal, G.: The final rifting evolution at deep magma-poor passive margins from Iberia-Newfoundland: a new point of view, Int. J. Earth Sci., 98, 1581–1597, https://doi.org/10.1007/s00531-008-0337-9, 2009. a, b
Peron-Pinvidic, G., Manatschal, G., and The “imagining Rifting” Workshop Participants: Rifted Margins: State of the Art and Future Challenges, Front. Earth Sci., 7, 218, https://doi.org/10.3389/feart.2019.00218, 2019. a, b
Petit, C., Déverchère, J., Houdry, F., Sankov, V. A., Melnikova, V. I., and Delvaux, D.: Present-day stress field changes along the Baikal rift and tectonic implications, Tectonics, 15, 1171–1191, https://doi.org/10.1029/96tc00624, 1996. a
Phethean, J. J. J., Papadopoulou, M., and Peace, A. L.: Dense melt residues drive mid-ocean-ridge “hotspots”, in: In the Footsteps of Warren B. Hamilton: New Ideas in Earth Science, Geological Society of America, https://doi.org/10.1130/2021.2553(30), 2022. a
Pirajno, F., Uysal, I., and Naumov, E. A.: Oceanic lithosphere and ophiolites: Birth, life and final resting place of related ore deposits, Gondwana Res., 88, 333–352, https://doi.org/10.1016/j.gr.2020.08.004, 2020. a, b
Poppe, S., Gilchrist, J. T., Breard, E. C. P., Graettinger, A., and Pansino, S.: Analog experiments in volcanology: towards multimethod, upscaled, and integrated models, B. Volcanol., 84, 52, https://doi.org/10.1007/s00445-022-01543-x, 2022. a
Pouclet, A. and Bram, K.: Nyiragongo and Nyamuragira: a review of volcanic activity in the Kivu rift, western branch of the East African Rift System, B. Volcanol., 83, 10, https://doi.org/10.1007/s00445-021-01435-6, 2021. a
Pudasainee, D., Kurian, V., and Gupta, R.: 2 – Coal: Past, Present, and Future Sustainable Use, in: Future Energy, 3rd edn., edited by: Letcher, T. M., Elsevier, 21–48, https://doi.org/10.1016/B978-0-08-102886-5.00002-5, 2020. a
Radhakrishna, T., Mohamed, A. R., Venkateshwarlu, M., Soumya, G. S., and Prachiti, P. K.: Mechanism of rift flank uplift and escarpment formation evidenced by Western Ghats, India, Sci. Rep.-UK, 9, 10511, https://doi.org/10.1038/s41598-019-46564-3, 2019. a
Regorda, A., Thieulot, C., van Zelst, I., Erdős, Z., Maia, J., and Buiter, S.: Rifting Venus: Insights from numerical modeling, J. Geophys. Res.-Planet., 128, e2022JE007588, https://doi.org/10.1029/2022je007588, 2023. a
Ridley, J.: Ore Deposit Geology, Cambridge University Press, https://doi.org/10.1017/CBO9781139135528, 2013. a, b, c, d, e, f, g, h
Rime, V., Foubert, A., Ruch, J., and Kidane, T.: Tectonostratigraphic evolution and significance of the Afar Depression, Earth-Sci. Rev., 244, 104519, https://doi.org/10.1016/j.earscirev.2023.104519, 2023. a, b, c
Robb, L.: Introduction to Ore-Forming Processes, Wiley, 496, pp., https://www.wiley.com/en-ca/Introduc4on+to+Ore-Forming+Processes'+2nd+Edi4on-p-9781119967507#evalua4on-copy-sec4on (last access: 11 July 2024), 2004. a
Roberts, D. G. and Bally, A. W. (Eds.): Regional Geology and Tectonics: Phanerozoic Passive Margins, Cratonic Basins and Global Tectonic Maps, Elsevier, https://doi.org/10.1016/C2010-0-67672-3, 2012. a
Rodríguez, A., Weis, P., Magnall, J. M., and Gleeson, S. A.: Hydrodynamic constraints on ore formation by basin-scale fluid flow at continental margins: Modelling Zn metallogenesis in the Devonian Selwyn Basin, Geochem. Geophy. Geosy., 22, e2020GC009453, https://doi.org/10.1029/2020gc009453, 2021. a
Romm, J.: A new forerunner for continental drift, Nature, 367, 407–408, https://doi.org/10.1038/367407a0, 1994. a, b
Rossello, E. A., Heit, B., and Bianchi, M.: Shallow intraplate seismicity in the Buenos Aires province (Argentina) and surrounding areas: is it related to the Quilmes Trough?, Boletín de Geología, 42, 31–48, https://doi.org/10.18273/revbol.v42n2-2020002, 2020. a
Rotevatn, A., Jackson, C. A., Tvedt, A. B. M., Bell, R. E., and Blækkan, I.: How do normal faults grow?, J. Struct. Geol., 125, 174–184, https://doi.org/10.1016/j.jsg.2018.08.005, 2019. a
Rowan, M. G.: Passive-margin salt basins: hyperextension, evaporite deposition, and salt tectonics, Basin Res., 26, 154–182, https://doi.org/10.1111/bre.12043, 2014. a, b
Ruppel, C. D. and Kessler, J. D.: The interaction of climate change and methane hydrates, Rev. Geophys., 55, 126–168, https://doi.org/10.1002/2016RG000534, 2017. a, b
Russell, M. J., Hall, A. J., and Martin, W.: Serpentinization as a source of energy at the origin of life, Geobiology, 8, 355–371, https://doi.org/10.1111/j.1472-4669.2010.00249.x, 2010. a
Ryan, W. B. F., Carbotte, S. M., Coplan, J. O., O'Hara, S., Melkonian, A., Arko, R., Weissel, R. A., Ferrini, V., Goodwillie, A., Nitsche, F., Bonczkowski, J., and Zemsky, R.: Global multi-resolution topography synthesis, Geochem. Geophy. Geosy., 10, Q03014, https://doi.org/10.1029/2008gc002332, 2009. a, b
Salgado, A. A. R., de Andrade Rezende, E., Bourlès, D., Braucher, R., da Silva, J. R., and Garcia, R. A.: Relief evolution of the Continental Rift of Southeast Brazil revealed by in situ-produced 10Be concentrations in river-borne sediments, J. S. Am. Earth Sci., 67, 89–99, https://doi.org/10.1016/j.jsames.2016.02.002, 2016. a
Samsu, A., Micklethwaite, S., Williams, J., Fagereng, A., and Cruden, A. R.: A review of structural inheritance in rift basin formation, https://eartharxiv.org/repository/view/4665/ (last access: 11 July 2024), 2022. a
Sanner, B.: Baden-Baden – A Famous Thermal Spa with a Long History, GHC Bulletin, 16–22, https://citeseerx.ist.psu.edu/document?repid=rep1&type=pdf&doi=a3b86e6cf62727d67c723e4e2b471c762211f26e (last access: 11 July 2024), 2000. a
Sapin, F., Ringenbach, J.-C., and Clerc, C.: Rifted margins classification and forcing parameters, Sci. Rep.-UK, 11, 8199, https://doi.org/10.1038/s41598-021-87648-3, 2021. a, b, c
Sawamura, P., Vernier, J. P., Barnes, J. E., Berkoff, T. A., Welton, E. J., Alados-Arboledas, L., Navas-Guzmán, F., Pappalardo, G., Mona, L., Madonna, F., Lange, D., Sicard, M., Godin-Beekmann, S., Payen, G., Wang, Z., Hu, S., Tripathi, S. N., Cordoba-Jabonero, C., and Hoff, R. M.: Stratospheric AOD after the 2011 eruption of Nabro volcano measured by lidars over the Northern Hemisphere, Environ. Res. Lett., 7, 034013, https://doi.org/10.1088/1748-9326/7/3/034013, 2012. a
Sawyer, G. M., Oppenheimer, C., Tsanev, V. I., and Yirgu, G.: Magmatic degassing at Erta 'Ale volcano, Ethiopia, J. Volcanol. Geoth. Res., 178, 837–846, https://doi.org/10.1016/j.jvolgeores.2008.09.017, 2008. a
Schiffer, C., Doré, A. G., Foulger, G. R., Franke, D., Geoffroy, L., Gernigon, L., Holdsworth, B., Kusznir, N., Lundin, E., McCaffrey, K., Peace, A. L., Petersen, K. D., Phillips, T. B., Stephenson, R., Stoker, M. S., and Welford, J. K.: Structural inheritance in the North Atlantic, Earth-Sci. Rev., 206, 102975, https://doi.org/10.1016/j.earscirev.2019.102975, 2020. a
Schmid, M., Tietze, K., Halabwachs, M., Lorke, A., McGinnis, D., and Wüest, A.: How hazardous is the gas accumulation in Lake Kivu? Arguments for a risk assessment in light of the Nyiragongo Volcano eruption of 2002, Acta Vulcanologica, 14, 115–122, 2002. a
Schneider, G. I. C.: Marine diamond mining in the Benguela Current Large Marine Ecosystem: The case of Namibia, Environmental Development, 36, 100579, https://doi.org/10.1016/j.envdev.2020.100579, 2020. a
Schulte, S. M. and Mooney, W. D.: An updated global earthquake catalogue for stable continental regions: reassessing the correlation with ancient rifts, Geophys. J. Int., 161, 707–721, https://doi.org/10.1111/j.1365-246X.2005.02554.x, 2005. a
Schulz, H.-M., Horsfield, B., and Sachsenhofer, R. F.: Shale gas in Europe: a regional overview and current research activities, Geological Society, London, Petroleum Geology Conference Series, 7, 1079–1085, https://doi.org/10.1144/0071079, 2010. a
Sekajugo, J., Kagoro-Rugunda, G., Mutyebere, R., Kabaseke, C., Namara, E., Dewitte, O., Kervyn, M., and Jacobs, L.: Can citizen scientists provide a reliable geo-hydrological hazard inventory? An analysis of biases, sensitivity and precision for the Rwenzori Mountains, Uganda, Environ. Res. Lett., 17, 045011, https://doi.org/10.1088/1748-9326/ac5bb5, 2022. a
Şengör, A. M. C.: Continental Rifts, in: Encyclopedia of Solid Earth Geophysics, edited by: Gupta, H. K., Springer International Publishing, Cham, 1–15, https://doi.org/10.1007/978-3-030-10475-7_31-2, 2020. a, b, c
Şengör, A. M. C. and Natal'in, B. A.: Rifts of the world, in: Mantle plumes: their identification through time, vol. 352, edited by: Ernst, R. E. and Buchan, K. L., Geological Society of America, https://doi.org/10.1130/0-8137-2352-3.389, 2001. a
Seton, M., Müller, R. D., Zahirovic, S., Williams, S., Wright, N. M., Cannon, J., Whittaker, J. M., Matthews, K. J., and McGirr, R.: A Global Data Set of Present-Day Oceanic Crustal Age and Seafloor Spreading Parameters, Geochem. Geophy. Geosy., 21, e2020GC009214, https://doi.org/10.1029/2020GC009214, 2020. a
Shanks III, W. C. P., Koski, R. A., Mosier, D. L., Schulz, K. J., Morgan, L. A., Slack, J. F., Ridley, W. I., Dusel-Bacon, C., Seal II, R. R., and Piatak, N. M.: Volcanogenic massive sulfide occurrence model: Chapter C in Mineral deposit models for resource assessment, Tech. Rep. 2010-5070C, U. S. Geological Survey, Reston, VA, https://doi.org/10.3133/sir20105070C, 2012. a
Sharma, U., Ray, Y., and Pandey, M.: Topography and rainfall coupled landscape evolution of the passive margin of Sahyadri (Western Ghats), India, Geosystems and Geoenvironment, 1, 100100, https://doi.org/10.1016/j.geogeo.2022.100100, 2022. a
Sheldon, H. A., Schaubs, P. M., Blaikie, T. N., Kunzmann, M., Schmid, S., and Spinks, S.: An integrated study of the McArthur River mineral system: From geochemistry, geophysics and sequence stratigraphy to basin-scale models of fluid flow, in: Annual Geoscience Exploration Seminar (AGES) Proceedings, Northern Territory Geological Survey, 81, https://geoscience.nt.gov.au/gemis/ntgsjspui/handle/1/88379 (last access: 11 July 2024), 2019. a
Skopljak, N.: History written offshore Denmark: First CO2 storage in the North Sea, https://www.offshore-energy.biz/history-written-offshore-denmark-first-co2-storage-in-the-north-sea/, (last access: 23 October 2023), 2023. a
Smith, M. P., Campbell, L. S., and Kynicky, J.: A review of the genesis of the world class Bayan Obo Fe–REE–Nb deposits, Inner Mongolia, China: Multistage processes and outstanding questions, Ore Geol. Rev., 64, 459–476, https://doi.org/10.1016/j.oregeorev.2014.03.007, 2015. a
Smith, N. J. P., Shepherd, T. J., Styles, M. T., and Williams, G. M.: Hydrogen exploration: a review of global hydrogen accumulations and implications for prospective areas in NW Europe, Geological Society, London, Petroleum Geology Conference Series, 6, 349–358, https://doi.org/10.1144/0060349, 2005. a
Snæbjörnsdóttir, S. Ó., Sigfússon, B., Marieni, C., Goldberg, D., Gislason, S. R., and Oelkers, E. H.: Carbon dioxide storage through mineral carbonation, Nature Reviews Earth & Environment, 1, 90–102, https://doi.org/10.1038/s43017-019-0011-8, 2020. a, b
Snedden, J. W. and Galloway, W. E.: The Gulf of Mexico Sedimentary Basin: Depositional Evolution and Petroleum Applications, Cambridge University Press, https://doi.org/10.1017/9781108292795, 2019. a
Sobolik, S. R., Hart, D., Chojnicki, K., and Park, B.: 2019 Annual Report of Available Drawdowns for Each Oil Storage Cavern in the Strategic Petroleum Reserve, Tech. Rep. SAND-2019-3673, Sandia National Lab. (SNL-NM), Albuquerque, NM (United States), https://doi.org/10.2172/1762083, 2019. a
Stamps, D. S., Kreemer, C., Fernandes, R., Rajaonarison, T. A., and Rambolamanana, G.: Redefining East African Rift System kinematics, Geology, 49, 150–155, https://doi.org/10.1130/G47985.1, 2021. a, b
Stanley, T. and Kirschbaum, D. B.: A heuristic approach to global landslide susceptibility mapping, Nat. Hazards, 87, 145–164, https://doi.org/10.1007/s11069-017-2757-y, 2017. a
Steiner, B. M.: W and Li-Cs-Ta geochemical signatures in I-type granites – A case study from the Vosges Mountains, NE France, J. Geochem. Explor., 197, 238–250, https://doi.org/10.1016/j.gexplo.2018.12.009, 2019. a
Stober, I. and Bucher, K.: Geothermal Energy, Springer International Publishing, https://doi.org/10.1007/978-3-030-71685-1, 2021. a
Stockman, S., Lawson, D. J., and Werner, M. J.: Forecasting the 2016–2017 Central Apennines earthquake sequence with a neural point process, Earth's Future, 11, e2023EF003777, https://doi.org/10.1029/2023ef003777, 2023. a
Styron, R. and Pagani, M.: The GEM Global Active Faults Database, Earthq. Spectra, 36, 160–180, https://doi.org/10.1177/8755293020944182, 2020. a, b
Suhr, B. and Six, K.: Simple particle shapes for DEM simulations of railway ballast: influence of shape descriptors on packing behaviour, Granul. Matter, 22, 43, https://doi.org/10.1007/s10035-020-1009-0, 2020. a
Tarkowski, R.: Underground hydrogen storage: Characteristics and prospects, Renew. Sust. Energ. Rev., 105, 86–94, https://doi.org/10.1016/j.rser.2019.01.051, 2019. a
Tarkowski, R., Uliasz-Misiak, B., and Tarkowski, P.: Storage of hydrogen, natural gas, and carbon dioxide – Geological and legal conditions, Int. J. Hydrogen Energ., 46, 20010–20022, https://doi.org/10.1016/j.ijhydene.2021.03.131, 2021. a, b
Tassi, F. and Rouwet, D.: An overview of the structure, hazards, and methods of investigation of Nyos-type lakes from the geochemical perspective, J. Limnol., 73, 55–70, https://doi.org/10.4081/jlimnol.2014.836, 2014. a
Taylor, B., Goodliffe, A. M., and Martinez, F.: How continents break up: Insights from Papua New Guinea, J. Geophys. Res., 104, 7497–7512, https://doi.org/10.1029/1998jb900115, 1999. a
Taylor, C. D., Schulz, K. J., Doebrich, J. L., Orris, G., Denning, P., and Kirschbaum, M. J.: Geology and Nonfuel Mineral Deposits of Africa and the Middle East, Tech. Rep. 2005-1294-E, U. S. Geological Survey, https://doi.org/10.3133/ofr20051294E, 2009. a
Thingbaijam, K. K. S., Martin Mai, P., and Goda, K.: New empirical earthquake source-scaling laws, B. Seismol. Soc. Am., 107, 2225–2246, https://doi.org/10.1785/0120170017, 2017. a
Thran, M. C., Brune, S., Webster, J. M., Dominey-Howes, D., and Harris, D.: Examining the impact of the Great Barrier Reef on tsunami propagation using numerical simulations, Nat. Hazards, 108, 347–388, https://doi.org/10.1007/s11069-021-04686-w, 2021. a
TNO: BROloket, https://www.broloket.nl/ondergrondgegevens (last access: 15 March 2024), 2024a. a
TNO: DINOloket, https://www.dinoloket.nl/en (last access: 15 March 2024), 2024b. a
Tucker, O. D.: Carbon Capture and Storage, IOP Publishing Limited, https://doi.org/10.1088/978-0-7503-1581-4, 2018. a, b, c
Tugend, J., Manatschal, G., Kusznir, N. J., and Masini, E.: Characterizing and identifying structural domains at rifed continental margins: application to the Bay of Biscay margins and its Western Pyrenean fossil remnants, Geol. Soc. Lond. Spec. Publ., 413, 171–203, https://doi.org/10.1144/SP413.3, 2015. a
Turner, J. P., Berry, T. W., Bowman, M. J., and Chapman, N. A.: Role of the geosphere in deep nuclear waste disposal – An England and Wales perspective, Earth-Sci. Rev., 242, 104445, https://doi.org/10.1016/j.earscirev.2023.104445, 2023. a
UNEP: Annual Report 2022, Tech. rep., UN Environment Programme, https://www.unep.org/resources/annual-report-2022 (last access: 11 July 2024), 2023. a
USDC: A Federal Strategy to Ensure Secure and Reliable Supplies of Critical Minerals, Tech. rep., U.S. Department of Commerce, https://www.commerce.gov/data-and-reports/reports/2019/06/federal-strategy-ensure-secure-and-reliable-supplies-critical-minerals (last access: 11 July 2024), 2019. a
van der Elst, N. J., Savage, H. M., Keranen, K. M., and Abers, G. A.: Enhanced remote earthquake triggering at fluid-injection sites in the midwestern United States, Science, 341, 164–167, https://doi.org/10.1126/science.1238948, 2013. a, b
van Wijk, J. W. and Cloetingh, S. A. P. L.: Basin migration caused by slow lithospheric extension, Earth Planet. Sc. Lett., 198, 275–288, https://doi.org/10.1016/S0012-821X(02)00560-5, 2002. a
Varet, J.: Geology of Afar (East Africa), Springer Cham, https://doi.org/10.1007/978-3-319-60865-5, 2018. a, b, c, d, e, f
Venzke, E.: Volcanoes of the World, https://doi.org/10.5479/si.GVP.VOTW5-2023.5.1, 2023. a
Vereb, V., van Wyk de Vries, B., Guilbaud, M.-N., and Karátson, D.: The Urban Geoheritage of Clermont-Ferrand: From Inventory to Management, Quaestiones Geographicae, 39, 5–31, https://doi.org/10.2478/quageo-2020-0020, 2020. a
Walle, H., Zewde, S., and Heldal, T.: Building stone of central and southern Ethiopia: deposits and resource potential, NGU-BULL, 436, 175–182, https://www.ngu.no/upload/Publikasjoner/Bulletin/Bulletin436_175-182.pdf (last access: 11 July 2024), 2000. a
Walsh, J. J., Torremans, K., Güven, J., Kyne, R., Conneally, J., and Bonson, C.: Fault-Controlled Fluid Flow Within Extensional Basins and Its Implications for Sedimentary Rock-Hosted Mineral Deposits, in: Metals, Minerals, and Society, vol. 21, edited by: Arribas R., A. M. and Mauk, J. L., Society of Economic Geologists (SEG), https://doi.org/10.5382/SP.21.11, 2018. a
Wang, L., Maestrelli, D., Corti, G., Zou, Y., and Shen, C.: Normal fault reactivation during multiphase extension: Analogue models and application to the Turkana depression, East Africa, Tectonophysics, 811, 228870, https://doi.org/10.1016/j.tecto.2021.228870, 2021. a
Wangen, M.: The blanketing effect in sedimentary basins, Basin Res., 7, 283–298, https://doi.org/10.1111/j.1365-2117.1995.tb00118.x, 1995. a
Warsitzka, M., Závada, P., Jähne-Klingberg, F., and Krzywiec, P.: Contribution of gravity gliding in salt-bearing rift basins – a new experimental setup for simulating salt tectonics under the influence of sub-salt extension and tilting, Solid Earth, 12, 1987–2020, https://doi.org/10.5194/se-12-1987-2021, 2021. a, b
Washburn, T. W., Turner, P. J., Durden, J. M., Jones, D. O. B., Weaver, P., and Van Dover, C. L.: Ecological risk assessment for deep-sea mining, Ocean Coast. Manage., 176, 24–39, https://doi.org/10.1016/j.ocecoaman.2019.04.014, 2019. a
Watters, T. R., Montési, L. G. J., Oberst, J., and Preusker, F.: Fault-bound valley associated with the Rembrandt basin on Mercury, Geophys. Res. Lett., 43, 11536–11544, https://doi.org/10.1002/2016gl070205, 2016. a
Weert, A., Ogata, K., Vinci, F., Leo, C., Bertotti, G., Amory, J., and Tavani, S.: Multiple phase rifting and subsequent inversion in the West Netherlands Basin: implications for geothermal reservoir characterization, EGUsphere [preprint], https://doi.org/10.5194/egusphere-2023-1126, 2023. a
Wenz, J.: The danger lurking in an African lake, Knowable magazine, https://doi.org/10.1146/knowable-100720-1, 2020. a
Wernicke, B.: Uniform-sense normal simple shear of the continental lithosphere, Can. J. Earth Sci., 22, 108–125, https://doi.org/10.1139/e85-009, 1985. a
White, R. S., Hardman, R. F. P., Watts, A. B., Whitmarsh, R. B., Lambiase, J. J., and Morley, C. K.: Hydrocarbons in rift basins: the role of stratigraphy, Philos. T. Roy. Soc. A, 357, 877–900, https://doi.org/10.1098/rsta.1999.0356, 1999. a
Whittaker, S., Rostron, B., Hawkes, C., Gardner, C., White, D., Johnson, J., Chalaturnyk, R., and Seeburger, D.: A decade of CO2 injection into depleting oil fields: Monitoring and research activities of the IEA GHG Weyburn-Midale CO2 Monitoring and Storage Project, Enrgy. Proced., 4, 6069–6076, https://doi.org/10.1016/j.egypro.2011.02.612, 2011. a
Wichman, B. G. H. M., Knoeff, J. G., and de Vries, G.: Geotechnical aspects of reinforcement alternatives of the Afsluitdijk, in: Proceedings of the 17th International Conference on Soil Mechanics and Geotechnical Engineering, vols. 1, 2, 3 and 4, IOS Press, Amsterdam, NY, 1514–1517, https://doi.org/10.3233/978-1-60750-031-5-1514, 2009. a
Wiener, R. W., Mann, M. G., Angelich, M. T., and Molyneux, J. B.: Mobile Shale in the Niger Delta: Characteristics, Structure, and Evolution, in: Shale Tectonics, vol. 93, edited by: Wood, L. J., American Association of Petroleum Geologists, https://doi.org/10.1306/13231313M933423, 2011. a
Wilkinson, J. J.: 13.9 – Sediment-Hosted Zinc–Lead Mineralization: Processes and Perspectives, in: Treatise on Geochemistry, 2nd edn., edited by: Holland, H. D. and Turekian, K. K., Elsevier, Oxford, 219–249, https://doi.org/10.1016/B978-0-08-095975-7.01109-8, 2014. a, b, c
Williams, J. N., Wedmore, L. N. J., Fagereng, Å., Werner, M. J., Mdala, H., Shillington, D. J., Scholz, C. A., Kolawole, F., Wright, L. J. M., Biggs, J., Dulanya, Z., Mphepo, F., and Chindandali, P.: Geologic and geodetic constraints on the magnitude and frequency of earthquakes along Malawi's active faults: the Malawi Seismogenic Source Model (MSSM), Nat. Hazards Earth Syst. Sci., 22, 3607–3639, https://doi.org/10.5194/nhess-22-3607-2022, 2022. a, b
Williams Jr., R. S. and Moore, J. G.: Man against volcano: The eruption on Heimaey, Vestmann Islands, Iceland, USGS, https://doi.org/10.3133/70039211, 1976. a
Wilson, C. J. N., Blake, S., Charlier, B. L. A., and Sutton, A. N.: The 26·5 ka oruanui eruption, taupo volcano, New Zealand: Development, characteristics and evacuation of a large rhyolitic magma body, J. Petrol., 47, 35–69, https://doi.org/10.1093/petrology/egi066, 2006. a
Wilson, J. T.: Static or Mobile Earth: The Current Scientific Revolution, P. Am. Philos. Soc., 112, 309–320, 1968. a, b
Wilson, R. W., Houseman, G. A., Buiter, S. J. H., McCaffrey, K. J. W., and Doré, A. G.: Fifty years of the Wilson Cycle concept in plate tectonics: an overview, Geol. Soc. Lond. Spec. Publ., 470, 1–17, https://doi.org/10.1144/SP470-2019-58, 2019. a, b
Wilson, T. J.: Did the Atlantic Close and then Re-Open?, Nature, 211, 676–681, https://doi.org/10.1038/211676a0, 1966. a, b
Wolfenden, E., Ebinger, C., Yirgu, G., Renne, P. R., and Kelley, S. P.: Evolution of a volcanic rifted margin: Southern Red Sea, Ethiopia, GSA Bulletin, 117, 846–864, https://doi.org/10.1130/B25516.1, 2005. a
Wölfl, A.-C., Snaith, H., Amirebrahimi, S., Devey, C. W., Dorschel, B., Ferrini, V., Huvenne, V. A. I., Jakobsson, M., Jencks, J., Johnston, G., Lamarche, G., Mayer, L., Millar, D., Pedersen, T. H., Picard, K., Reitz, A., Schmitt, T., Visbeck, M., Weatherall, P., and Wigley, R.: Seafloor mapping – the challenge of a truly global ocean bathymetry, Frontiers in Marine Science, 6, 283, https://doi.org/10.3389/fmars.2019.00283, 2019. a
Worldometer: Population of eastern Africa (2023) – Worldometer, https://www.worldometers.info/world-population/eastern-africa-population/ (last access: 23 October 2023), 2023. a
Xu, Y., He, H., Deng, Q., Allen, M. B., Sun, H., and Bi, L.: The CE 1303 Hongdong Earthquake and the Huoshan Piedmont Fault, Shanxi Graben: Implications for Magnitude Limits of Normal Fault Earthquakes, J. Geophys. Res.-Sol. Ea., 123, 3098–3121, https://doi.org/10.1002/2017JB014928, 2018. a
Yamamoto, K., Boswell, R., Collett, T. S., Dallimore, S. R., and Lu, H.: Review of Past Gas Production Attempts from Subsurface Gas Hydrate Deposits and Necessity of Long-Term Production Testing, Energ. Fuel., 36, 5047–5062, https://doi.org/10.1021/acs.energyfuels.1c04119, 2022. a
Yang, Z. and Chen, W.-P.: Earthquakes along the East African Rift System: A multiscale, system-wide perspective, J. Geophys. Res., 115, B12309, https://doi.org/10.1029/2009jb006779, 2010. a, b
Yaxley, G. M., Anenburg, M., Tappe, S., Decree, S., and Guzmics, T.: Carbonatites: Classification, Sources, Evolution, and Emplacement, Annu. Rev. Earth Pl. Sc., 50, 261–293, https://doi.org/10.1146/annurev-earth-032320-104243, 2022. a
Zappettini, E. O., Rubinstein, N., Crosta, S., and Segal, S. J.: Intracontinental rift-related deposits: A review of key models, Ore Geol. Rev., 89, 594–608, https://doi.org/10.1016/j.oregeorev.2017.06.019, 2017. a, b
Zhang, J., Li, Z., Wang, D., Xu, L., Li, Z., Niu, J., Chen, L., Sun, Y., Li, Q., Yang, Z., Zhao, X., Wu, X., and Lang, Y.: Shale gas accumulation patterns in China, Natural Gas Industry B, 10, 14–31, https://doi.org/10.1016/j.ngib.2023.01.004, 2023. a
Zhong, R., Brugger, J., Chen, Y., and Li, W.: Contrasting regimes of Cu, Zn and Pb transport in ore-forming hydrothermal fluids, Chem. Geol., 395, 154–164, https://doi.org/10.1016/j.chemgeo.2014.12.008, 2015. a
Zhou, F., Dyment, J., Tao, C., and Wu, T.: Magmatism at oceanic core complexes on the ultraslow Southwest Indian Ridge: Insights from near-seafloor magnetics, Geology, 50, 726–730, https://doi.org/10.1130/G49771.1, 2022. a
Zielke, O. and Strecker, M. R.: Recurrence of Large Earthquakes in Magmatic Continental Rifts: Insights from a Paleoseismic Study along the Laikipia–Marmanet Fault, Subukia Valley, Kenya Rift, B. Seismol. Soc. Am., 99, 61–70, https://doi.org/10.1785/0120080015, 2009. a
Zlydenko, O., Elidan, G., Hassidim, A., Kukliansky, D., Matias, Y., Meade, B., Molchanov, A., Nevo, S., and Bar-Sinai, Y.: A neural encoder for earthquake rate forecasting, Sci. Rep.-UK, 13, 12350, https://doi.org/10.1038/s41598-023-38033-9, 2023. a
Zwaan, F. and Schreurs, G.: The link between Somalian Plate rotation and the East African Rift System: an analogue modelling study, Solid Earth, 14, 823–845, https://doi.org/10.5194/se-14-823-2023, 2023. a
Zwaan, F., Corti, G., Sani, F., Keir, D., Muluneh, A. A., Illsley-Kemp, F., and Papini, M.: Structural analysis of the western afar margin, east Africa: Evidence for multiphase rotational rifting, Tectonics, 39, e2019TC006043, https://doi.org/10.1029/2019tc006043, 2020. a
Zwaan, F., Chenin, P., Erratt, D., Manatschal, G., and Schreurs, G.: Competition between 3D structural inheritance and kinematics during rifting: Insights from analogue models, Basin Res., 34, 824–854, https://doi.org/10.1111/bre.12642, 2022. a, b, c, d
Zwaan, F., Brune, S., Glerum, A., Vasey, D. A., Naliboff, J. B., Manatschal, G., and Gaucher, E. C.: Rift-inversion orogens are potential hotspots for natural H2 generation, ResearchSquare, https://doi.org/10.21203/rs.3.rs-3367317/v1, 2023. a, b